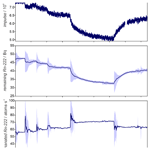
Radon metrology for use in climate change observation and radiation protection at the environmental level
Annette Röttger
Claudia Grossi
Arturo Vargas
Ute Karstens
Giorgia Cinelli
Edward Chung
Dafina Kikaj
Chris Rennick
Florian Mertes
Ileana Radulescu
Radon (222Rn) gas is the largest source of public exposure to naturally occurring radioactivity and the identification of radon priority areas is required by the Council Directive 2013/59/Euratom. Radon is also used as a tracer to improve atmospheric transport models and to indirectly estimate greenhouse gas (GHG) fluxes using the Radon Tracer Method (RTM). This method is based on the correlation between atmospheric concentrations of radon and GHG, together with information on the radon flux data. For radiological data, all European countries have installed networks of automatic gamma dose rate monitoring stations and report the real-time information gathered to the European Radiological Data Exchange Platform (EURDEP). So far, atmospheric radon activity concentrations and radon fluxes are not yet reported in EURDEP, nor routinely measured within the European radiological networks although these observations could help to avoid false positives results.
Due to above applications, there is a need of building a metrological chain to ensure high quality radon activity concentrations and radon flux measurements. Both climate and radiation protection research communities underline the need for improved traceability in low-level atmospheric radon measurements (Khanbabaee et al., 2021). The EMPIR project 19ENV01 traceRadon1 is aimed towards providing the necessary measurement infrastructure and transfer standards to fulfil this need.
Results of this project are particularly important for improving independent GHG emission estimates that support national reporting under the Paris Agreement on climate change and for the Council Directive 2013/59/Euratom, thus benefitting two large scientific communities. In this paper, early results, such as new activity standard developments and an overview of commercial and research radon monitors are presented and discussed. These results will feed into the traceRadon project with respect to radionuclide metrology in air and its potential for the improvement of the RTM.
- Article
(264 KB) - Full-text XML
- BibTeX
- EndNote
The radioactive noble gas radon is a health hazard when accumulated indoor, from a radiation protection point of view. However, radon is being also studied in the environment as a useful tracer to investigate atmospheric processes, to improve Atmospheric Transport Models (ATM) or indirectly retrieve fluxes of greenhouse gases. To increase the comparability for both radiation protection measurements as well as those used for GHG modelling, traceability to the SI is needed for radon release rates from soil as well as its concentration in the atmosphere. Radon flux relates to the transfer process of radon activity from soil to the atmosphere per square meter and second (Bq m−2 s−1), whilst radon activity concentration is the amount of activity of radon in the atmosphere per cubic meter (Bq m−3). Traceability to a primary standard does not currently exist for these quantities, which limits the usability of their measurements and their harmonization across Europe.
Climatic Atmospheric Monitoring Networks (AMN) like the pan-European Integrated Carbon Observation System (ICOS, http://www.icos-cp.eu, last access: 2 March 2022), are infrastructures that operate GHG monitoring stations and are also including atmospheric radon measurements based on different techniques (Schmithüsen et al., 2017; Grossi et al., 2020). The radon data from these networks can be used, among others, to improve ATM, to study atmospheric processes and to indirectly estimate GHG fluxes by the Radon Tracer Method (RTM) (e.g., Van Der Laan et al., 2010; Levin et al., 2011, 2021; Vogel et al., 2012; Wada et al., 2013; Grossi et al., 2014, 2018), which uses the correlation between GHG and radon activity concentrations assuming a known radon flux over the footprint area. These measurements need significant improvement in terms of the accuracy of the environmental radon activity concentrations which ranges usually between 1 and 100 Bq m−3 to be able to provide robust data for the use in the RTM and thus minimize the uncertainties on the retrieved GHG fluxes. At present, commercial radon monitors currently available on the market present a high uncertainty when measuring radon activity concentration below 100 Bq m−3 as reported by Radulescu et al. (2022). Radon flux data, useful to validate radon flux maps and models, also need a robust traceability chain and a related infrastructure as explained in detail by Röttger et al. (2021).
Similarly, for radiation monitoring, all European countries have installed networks of automatic radiation dose and airborne contamination monitoring stations and report the information gathered to the EURDEP, thus supporting EU member states and the EURATOM treaty (Sangiorgi et al., 2020). Currently, monitoring information on dose rates is collected from 5500 automatic surveillance systems in 40 countries, however, urgently needed data on outdoor (atmospheric) radon activity concentrations is not yet collected due to a lack of harmonization between research monitors able to measure accurately at the low levels encountered in the environment. Furthermore, accurately detecting radioactivity from nuclear and radiological emergencies using ambient dose rate measurements relies on rejecting false positive results based on particulate radon progeny washed from the atmosphere by rain. Therefore, improving early warning detection systems for radioactivity requires greater accuracy in determining environmental radon activity concentrations.
The project traceRadon, started in June 2020 joining together the atmosphere, climate and the environmental radiation scientific communities under the umbrella of EURAMET, the European Association of National Metrology Institutes. At the very moment 18 partners and 12 collaborators are working towards a European harmonisation within the goals of this project, which consists of four technical Working Packages (WP):
-
WP1 aims to develop traceable methods for the measurement of outdoor low-level radon activity concentrations in the range of 1 to 100 Bq m−3 with uncertainties of 10 % (k=1) to be used in climate and radiation protection networks. These methods will include two new traceable 222Rn emanation sources below 100 Bq m−3, a traceable transfer instrument calibrated with these new sources and a calibration procedure suitable to enable a traceable calibration of environmental atmospheric radon measurement systems in the field;
-
WP2 aims to improve the accuracy of radon flux measurements for the purpose of: (1) identifying Radon Priority Areas (RPAs) for radiation protection goals; and (2) retrieving indirect GHG fluxes using the RTM. Within this work package four experimental measurement campaigns will be carried out at different European stations in order to produce high quality data to validate available radon flux models (Karstens et al., 2015) and inventories (Szegvary et al., 2009);
-
WP3 aims to validate current radon flux models and inventories using traceable measurements of radon flux and radon activity concentration supported by dosimetric and spectrometric data from the radiological early warning networks in Europe and, in addition, to improve process-based radon flux maps that can be used in the RTM, ATM and radiation protection;
-
WP4 will provide dynamic outdoor radon concentration and radon flux maps for climate change research and radiation protection. These last maps will be provided through the Radioactive Environmental Monitoring web portal (REMon, https://remon.jrc.ec.europa.eu, last access: 2 March 2022) operated by the Joint Research Centre of the European Commission and through the ICOS Carbon Portal (https://www.icos-cp.eu, last access: 2 March 2022). This new data will be linked to established data from EURDEP and EANR (https://remon.jrc.ec.europa.eu/About/Atlas-of-Natural-Radiation/Digital-Atlas, last access: 2 March 2022, Cinelli et al., 2019) and will be made available to scientists, policy makers and end users.
The project plan is laid out for 3 years and the work in the working packages is being performed in parallel. The project is managed by a coordinator and its results are disseminated by a dedicated impact work package. A social media account (@traceradon) is sharing the project news on a daily basis.
In the context of Earth system research, more detailed investigations of exchange processes of fluxes of GHG have increasingly come into focus in recent decades. Continuous measurements of GHG fluxes (CO2, CH4, N2O) between different ecosystems and the atmosphere are extremely heterogeneous because they are mainly dominated by local-scale changes in their sources and sinks, chemical reactions, as well as meteorological conditions. Local GHG flux measurements on the meter to kilometre scale are therefore often not representative of regional exchange rates on the scale of several kilometres. Here, the naturally occurring noble gas radon, which is emitted from continental soils but not from water bodies (such as oceans, seas, lakes, etc.) can help to overcome these difficulties by using it as a tracer for boundary layer mixing processes.
Similar to other gases which have distributed sources close to the ground radon accumulates, is mixed, and disperses within the atmospheric boundary layer. If the radon flux from the soil is known, the correlated increase of radon and GHG concentration can be used to estimate the GHG flux. Radon emissions from the land surface are influenced by the geophysical properties of the soils and its only sink is its radioactive decay (T1/2≈3.8 d). Radon is produced continuously by radioactive decay of radium (226Ra) in the soil, but its exhalation rate to the atmosphere is influenced by geophysical propriety of the soils (porosity, permeability, etc.) and by climatic conditions (rain, snow, temperature, etc.) (López-Coto et al., 2013; Karstens et al., 2015). Compared to the large spatiotemporal heterogeneity of GHG fluxes, radon emissions from ice-free land are often assumed to be more homogeneous (or at least uncorrelated with changes in the GHG fluxes on local spatial scales and diurnal time scales) and therefore easier to model. However, the reliability of the GHG flux estimates depend critically on the accuracy and representativeness of the value used for the radon flux in the footprint of the measurement station (Grossi et al., 2018; Levin et al., 2021) and hence requires a careful validation of the radon flux maps. Similarly, regional atmospheric transport models, such as those used in weather forecasting, can be validated and/or improved using radon as a transport tracer (e. g. Olivié et al., 2004; Koffi et al., 2016).
Within traceRadon, new chains of traceability are developed with respect to the activity concentration in the atmosphere as well as for the radon flux from the soil. Traceability in the metrological sense means that the measurement procedure is linked to the SI system of units in an absolute sense, in the expected measurement range (Röttger and Honig, 2011). An important part of the calibration and the measurement is the assignment of an uncertainty, which includes all individual uncertainty contributions (statistics of the measurement, uncertainty of the calibration, intrinsic background of the instrument, etc.). Because radon is so well suited as a tracer for climate monitoring, very sensitive radon monitors have been developed for this purpose during the last years by research entities and are running at different European atmospheric stations (Whittlestone and Zahorowski, 1998; Levin et al., 2002; Grossi et al., 2012; Griffiths et al., 2016). Sensitive means that, even at small activity concentrations down to few Bq m−3, small changes can be observed on an hourly basis. The ability to observe relative changes with a small uncertainty, however, says nothing about the uncertainty of the absolute value. The lack of traceability so far prevents radon measurements from being compared independently of the devices used at the different AMN stations as well as at radiological stations. Since the measuring devices are usually permanently installed at one location, the aim of the project is to develop a transportable calibration option for them.
The traceability for radon flux will not be covered by this publication in detail. But currently it can be stated that based on the observed needs, a radon “exhalation bed” to be used as a calibration facility has been designed and constructed. Several experiments were carried out with the aim for testing the reliability of an exhalation bed and to calibrate radon flux systems under different environmental conditions. Up to now different radon flux systems were simultaneously tested over the bed surface under dynamic or static conditions. The evaluation is not finished but shows promising results.
Atmospheric radon measurements are used in numerous atmospheric studies due to radon's unique physical and chemical characteristics (e.g., inert gas, simple source, and only radioactive decay as a sink). Among these applications, radon measurements in combination with GHG measurements can be used to indirectly estimate emission fluxes using the RTM (Dörr et al., 1983). The RTM uses atmospheric measurements of radon and the GHG of interest and measured, or modelled, values of radon fluxes. The RTM is based on the assumption that the nocturnal lower atmospheric boundary layer can be described as a well-mixed box of air where the changes in the radon and GHG concentrations are only due to their respective fluxes' contribution into the atmospheric volume. The change in atmospheric concentration conditions can be described as:
Where h(t) is the time dependent mixing height, ji(t) represents the surface flux of a species i, and ΔCi is the departure of its concentration relative to initial background levels for a given observation time Δt(i.e. t1−t0) The overbar indicates that both mixing height and net surface flux of the catchment area are averaged for the observation period. This version of the box model assumes a constant h−1 over the time window and neglects the radioactive decay of radon over the integration time period. Since the Eq. (1) can be obtained for both gases (e.g., 222Rn and GHG), the mixing layer height (which may be unknown) and time step cancel after taking the ratio yielding:
Where ΔCx and ΔC222Rn represent the concentration enhancements of species x (in this case one of the GHG) and radon above their respective background levels for a given observation period. The ‾jx and ‾jC222Rn represent the mean surface fluxes of 222Rn and GHG of the sources within the catchment footprint.
Radon flux, used for RTM applications in the literature, was usually taken as a constant value obtained by experimental measurements carried out close to the atmospheric station where radon and GHG atmospheric measurements were obtained or from the output of radon flux models in the grid containing the station location. Grossi et al. (2018) proposed for the first time to use within the RTM a potentially different “effective” radon flux influencing the atmospheric station each night of the studied time series. This value, changing for each night episode, was calculated by coupling the radon flux model, with the resident time map of the air masses over the region of interest. This last map was calculated using the backtrajectories with an atmospheric transport model (in their case the ECMWF-FLEXPART model (version 9.02) (Stohl et al., 1998)). This new methodology also accounts for the spatial variability of the radon flux within the footprint of the station which could be a significant problem for the RTM application as reported by Levin et al. (2021).
RTM is therefore based on both radon and GHG atmospheric concentration time series. However, so far RTM applications studies were carried out using: (1) local radon activity concentrations measured with different techniques and without being supported by a robust metrological traceability chain; (2) using radon flux value from not validated radon flux models or from experimental observations not supported by a proper petrology; (3) individual RTM without a specific application protocol. Thus, so far, GHG fluxes obtained by RTM at different locations and by different scientists cannot be compared because the RTM has not yet a unique application protocol. Firstly, there is a need to develop a traceability chain for radon instrument calibration from lab to field and secondly a standard protocol for RTM application is required where it should be specified, among others, the length of the nocturnal window to be analysed, the threshold of the linear correlation between radon and GHG during their increase, how to calculate the radon flux over the studied episodes. Both goals follow into the scope of the traceRadon project.
As described by Grossi et al. (2018) and Levin et al. (2021), it is very important to understand how the different selections in the application of this method will influence its result. Thus, there is a huge need to develop and test an agreed standard procedure for application of the RTM on different AMN sites. Such procedure would be a starting point for how the climate community could utilise radon measurements on local and regional scales from operational networks such as ICOS. The project traceRadon will provide main steps in overcoming these weaknesses. The first steps in this direction are described in section 6 and 7 by developing new sources and specifying requirements for transfer standards. Moreover, this procedure is important for GHG emission estimates that support national reporting under the Paris agreement on climate change.
The Council Directive 2013/59/EURATOM, published in 2014, contains new requirements for radon protection. Article 103 (3) requires Member States to identify areas where the radon activity concentration (annual mean) is expected to exceed the relevant national reference value in a significant number of buildings. In these areas, Member States are requested to establish programmes to carry out radon measurements in workplaces that are located at ground or basement level (Article 54 (2)). The establishment of Radon Priority Areas (RPA) is therefore an important step in the implementation of the Directive and in the radon action plans of the Member States.
Maps directly or indirectly related to soil 226Ra content existed in many member countries long before the implementation of the European Directive (Dubois, 2005). They are an important part of national radon strategies as a tool for prioritization of radon protection measures (e.g., structural precautions, radon measurements, awareness campaigns for decision makers and the public). Radon maps and RPAs can be produced and displayed using very different methods, depending on the intended use of the map and the available data (Bossew, 2018). Many maps and the setting of RPAs are based on indoor radon measurements in residential buildings, sometimes linked to information on building characteristics (e.g., Austria, see Friedmann, 2005). Other countries base the determination on data on radon in soil gas (e.g., Czech Republic and Germany, see Neznal et al., 2004 and Kemski et al., 2001) respectively. Another method for characterizing an area in terms of its radon potential is to link different radon-relevant parameters (e.g., radon in soil air, soil permeability, concentration of radionuclides in soil, soil characteristics). This is often referred to as the geogenic radon potential, and methods for this have been further developed thanks to the MetroRADON project (e.g., the Radon Hazard Index, RHI) (Bossew et al., 2020).
The Joint Research Centre of the European Commission (EC-JRC) has been collecting data on radon and natural sources of radiation from European countries and from available European databases. As results, the European Atlas of Natural Radiation (Tollefsen et al., 2019) contains a collection of maps showing the levels of natural sources of radiation in different parts of Europe. Moreover, it provides reference values for natural sources of radiation across Europe and makes harmonised datasets available to the scientific community and national authorities. This also serves the purpose of raising awareness about natural radioactivity in the public. The Atlas publication is available in digital format and as a printed version, in addition an online version is regularly updated when new data is available (https://remon.jrc.ec.europa.eu, last access: 2 March 2022). Radon outdoor air and radon flux maps have not been developed in the Atlas project so far, because the data were not yet available, and especially not robust and comparable. In the traceRadon project, for the first time an evaluation whether and how these data can be used in future methods and models for radon mapping and determination of RPAs is performed. Consideration of dynamic soil parameters (e.g., soil moisture) on radon transport processes, and thus on radon activity concentrations in soil air, may contribute significantly to characterization of radon potential. Within this project experimental calibrated data of atmospheric radon activity concentrations and radon flux as well as dose rate and spectrometry data (Röttger and Kessler, 2019) will be used to validate available radon flux models and inventories (e.g., Szegvary et al., 2009; Karstens et al., 2015). Finally, it is planned to make the radon outdoor air monitoring stations available online similar to the local gamma dose rate via EURDEP.
To start the development of new sources for the calibration needs (Linzmaier and Röttger, 2014), a literature study of currently available radon sources for the calibration of instruments capable of measuring radon activity concentrations below 100 Bq m−3 was performed. Environmental parameter ranges were evaluated and a suitability list for in-field calibration was defined. That led to new needs for these characteristics, specifically the ability to produce reference atmospheres at the ambient levels as well as high stability concerning environmental parameters for potential in-situ applications. Currently, no sources which meet both criteria are available and so alternative sources had to be developed for the traceRadon Project: low activity emanation sources. Two different principles were applied:
-
a radon emanation source is created from an emulsion of salts of fatty acids in silicone rubber, formed from a weighed standard solution of 226Ra. Traceability of the 226Ra activity is established by weighing and γ-spectrometry: Using a stainless-steel cylindrical case with valves and aerosol filters, applying ultra-dried air and a mass flow controller with additional humidifier, to control the dilution of the activity concentration, a time-stable radon activity concentration is achieved. This principal was already successfully applied in MetroRADON (Fialova et al., 2020);
-
a new development is the low-level, low activity emanation sources based on the electrodeposition from a carrier-free 226Ra solution on a stainless-steel plate. For futher information on this source design, the reader is directed to Mertes et al. (2020). The emanation rate of 222Rn of these sources is followed online via γ-spectrometry using portable scintillation detectors like LaBr3-Crystals.
Due to conservation of the amount of substance, the 222Rn activity retained within in an emanation source must balance the ingrowth, decay and release of 222Rn nuclei. Based on this observation, the measurement of residual 222Rn yields the emanation convolved with a certain impulse response characteristic that directly results from the well-known radioactive decay kinetics. Consequently, the γ-ray spectrometric measurement of the disequilibrium between 226Ra and the residual 222Rn decay products allows to directly calculate the emanation only once a stable state has been reached. During in-field calibrations, however, the environmental conditions are expected to change, which can change the emanation characteristics. To overcome this constraint, an algorithm implementing a technique of statistical inversion based on recursive Bayesian estimation has been developed and implemented (Mertes et al., 2021). Using this algorithm, the probability density function of the estimated emanation of 222Rn in terms of atoms per unit time is estimated using the supporting γ-ray spectrometric measurements. The recursive construction of the algorithm allows to best use the previously observed γ-ray spectra to determine the emanating amount in near real time, limited by the statistical uncertainty that can be achieved during the integration time of the spectrometer. As an input to the algorithm, the integral count-rate of a γ-ray spectrometer above 200 keV is used. A typical result of the application of this algorithm together with the input data is given in Fig. 1, which shows distinct behaviour of the inferred emanation resulting from changes in the relative humidity. Analogous behaviour is expected concerning changes in the ambient temperature and possibly pressure. Despite these environmental influences, different techniques of calibration can be realized based on the inference of a continuous 222Rn source term.
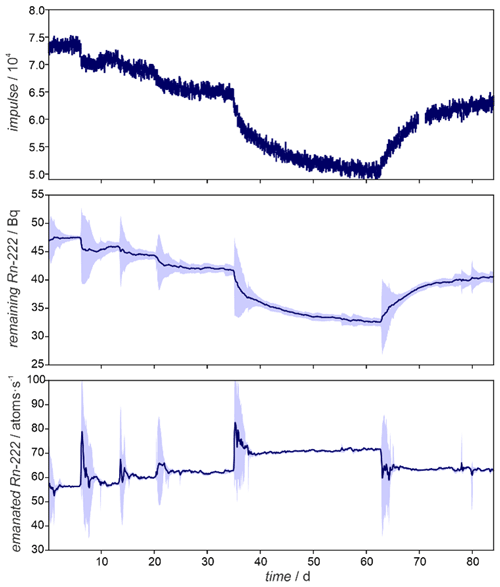
Figure 1The first (upper) diagram shows the integral count rate above the highest energy of 226Ra of an electrodeposited source, which is the input data to the algorithm. The variation of this count rate is achieved by variation of environmental parameters, specifically, the relative humidity. The second diagram presents the remaining 222Rn activity in the source, given in Bq, inferred from the count rate data. The light blue area represents the 90 % confidence interval for the uncertainty of the measurements. The third diagram gives the primary desired result, which is the emanated number of 222Rn atoms, represented as atoms s−1. Upon changes in the emanation, the confidence intervals increase, since the inference can then only be carried out using few data points. Within stable regimes, as time goes on, more data becomes available, such that the confidence intervals continually shrink.
In the scope of traceRadon, the two different sources will be compared regarding their applicability for in-situ calibrations, their stability with respect to environmental parameters as well as the match between their indicated 222Rn emanation values. Note, that the line of traceability for the two sources is very different: (1) Achieves traceability to the SI by the traceability of the weighing process and is thus traceable to the national 226Ra standards of the CMI (Fialova et al., 2020) and by characterization of the emanation by γ-ray spectrometry in comparison to simulated results. On the other hand, (2) achieves primary definition of the 226Ra activity, traceable to the meter and the second by employing defined solid-angle α-particle spectrometry and characterizes the emanation by γ-ray spectrometry in comparison with a sealed reference source (Mertes et al., 2020). While the stability with respect to environmental changes is assumed for source type (1), it can be at least retrospectively monitored for source type (2) using the outlined algorithm. Therefore, consistent calibration results with each source will provide significant confidence concerning the correctness of the applied methods. This may only be achieved by pursuing different source construction techniques with independent traceability chains.
With the new sources traceable calibrations are feasible, but as a prerequisite an instrument capable to operate in that range of activity concentration is needed. Nowadays a variety of commercial radon monitors are available on the market based on different measurement techniques. Additionally, radon monitors have also been developed at some research institutes. A literature review of the currently available continuous radon monitors capable of measuring activity concentrations below 100 Bq m−3 was carrying out during the first months of the traceRadon project. A summary of it is reported in Table 1 with the main characteristics of the monitors as declared by the manufacturer. Often the trade-off between commercial and research-grade radon monitors is a reduction of portability for an increase in accuracy at low-level measurements. Note that the nominal sensitivity in Table 1 is the one declared by the manufacturers and it is considering only the uncertainty of the total counts in the region of interest of each instrument for radon measurement and/or calculation. This information only gives a first order of magnitude to compare the different monitors but other factors influencing the total uncertainty of the measurement such as calibration factor uncertainty, background uncertainty, interference factor uncertainty, uncertainties of correction factors applied to the different monitors due to their measurement method (temperature, water content, pressure, time response, deconvolution, etc.) are not taken into account (Radulescu et al., 2022). In addition, a desired matrix of properties usable for radon activity concentration monitors for use as a transfer standard in the field are shown in Table 2. They also provide a mark to be accomplish for atmospheric radon measurements at AMN from the point of view of the traceRadon project. The matrix is available to guide developers and producers in instrumental design for climate observation networks, since currently no IEC standard for this application exists.
Atmospheric measurements of radon activity concentrations can become a pillar in the assessment and improvement of ATM. For this aim, the metrology of the radon activity concentration has been improved by the development of a new traceability chain in extension of Pierre et al. (2021), a new data analysis for the online source application and by the implementation of a matrix with recommended properties for the in-field application of a transfer standard radon monitor for atmospheric measurements.
This is also the first step to overcome the current weaknesses in the RTM. The next step within traceRadon will be to establish a traceability chain for radon flux measurements as well.
Useable dynamic radon activity concentration and radon flux maps will be provided through the Radioactive Environmental Monitoring web portal (REMon, https://remon.jrc.ec.europa.eu, last access: 2 March 2022) operated by the Joint Research Centre of the European Commission and through the ICOS Carbon Portal. This new data will be linked to already established data from EURDEP (European Radiological Data Exchange Platform) and EANR (European Atlas of Natural Radiation) and will be made available to scientists, policy makers and end users. Concerning radiation protection, the focus is on the improvement of methods for the identification of Radon Priority Areas and the identification of radon wash-out peaks from total gamma dose rate data.
The authors confirm that the data supporting the findings of this publication are available in the references given or in case of the emanation sources on the SharePoint of the tracRadon Project (https://npluk.sharepoint.com/sites/traceRadon, last access: 2 March 2022). Additional data on the emanation sources is available from the corresponding author upon reasonable request.
SR form PTB drafted the outline of the manuscript with contributions from all co-authors. The text was prepared by him and edited jointly (SR, AR, CG, AV, UK, GC, EC, DK, CR, FM, IR). All co-authors provided significant input according to their expertise, especially: SR is responsible for WP1, CG for WP2, UK manages WP3, GC handles WP4, AR is managing WP6, while the WP5 is in the responsibility of CR.
The contact author has declared that neither they nor their co-authors have any competing interests.
Publisher’s note: Copernicus Publications remains neutral with regard to jurisdictional claims in published maps and institutional affiliations.
This article is part of the special issue “Geoscience applications of environmental radioactivity (EGU21 GI6.2 session)”. It is a result of the EGU General Assembly 2021, 19–30 April 2021.
EMPIR 19ENV01 traceRadon started to run in summer 2020. It is supported by a broad global scientific community within atmospheric and climate research, radiation protection and metrology. All stakeholders are united by the goal of providing new and improved data and maps for science, the public and decision makers. The early results are coming from the design and building of new technologies for calibrating high-sensitivity atmospheric radon monitors. The consortium would like to thank the staff of project partners and collaborators of traceRadon, as well as all institutions and organisations that supported the project with recommendations. In the preparation of the project, the communication and discussion within EURADOS WG3 turned out to be very effective. Further thanks go to EURAMET e.V., the European Association of National Metrology Institutes which made such a project possible within the EMPIR framework program. For the time being, the project traceRadon has established the following collaborations by a Letter of Agreement (in the order of signature date): (1) Universität Heidelberg, Germany. (2) ANSTO, Australia's Nuclear Science and Technology Organisation, Australia. (3) ERA, European Radon Association, Europe. (4) Met Office, United Kingdom. (5) University of Novi Sad, Serbia. (6) Politecnico di Milano, Italy. (7) University of Cordoba, Spain. (8) EURADOS, e.V., Europe. (9) Universität Siegen, Germany. (10) IRSN, France. (11) ARPA Piemonte, Italy. (12) ARPA Valle d'Aosta, Italy. The consortium is grateful to have this powerful support from colleagues worldwide. Further collaboration interest is welcome.
This project traceRadon has received funding from the EMPIR programme co-financed by the Participating States and from the European Union's Horizon 2020 research and innovation programme. 19ENV01 traceRadon denotes the EMPIR project reference.
This project 19NET01 traceRadon has received funding from the EMPIR
programme co-financed by the Participating States and from the European
Union's Horizon 2020 research and innovation programme. 19NET01 traceRadon
denotes the EMPIR project reference.
This open-access publication was funded by the Physikalisch-Technische Bundesanstalt.
This paper was edited by Virginia Strati and reviewed by Alastair Williams and Xuemeng Chen.
Biraud, S., Ciais, P., Ramonet, M., Simmonds, P., Kazan, V., Monfray, P., O'Doherty, S., Spain, T., and Jennings, S.: European greenhouse gas emissions estimated from continuous atmospheric measurements and radon 222 at Mace Head, Ireland, J. Geophys. Res.-Atmos., 105, 1351–1366, https://doi.org/10.1029/1999JD900821, 2000.
Bossew P.: Radon priority areas – definition, estimation and uncertainty, Nuclear Technology and Radiation Protection, 33, 286–292, https://doi.org/10.2298/NTRP180515011B, 2018.
Bossew, P., Cinelli, G., Ciotoli, G., Crowley, Q., Cort, M., Medina, J., Gruber, V., Petermann, E., and Tollefsen, T.: Development of a geogenic radon hazard index – concept, history, experiences, Int. J. Environ. Res. Public Health, 17, 4134, https://doi.org/10.3390/ijerph17114134, 2020.
Brunke, E.-G., Labuschagne, C., Parker, B., van der Spuy, D., and Whittlestone, W.: Cape Point GAW Station 222Rn detector: factors affecting sensitivity and accuracy, Atmos. Environ., 36, 2257–2262, https://doi.org/10.1016/S1352-2310(02)00196-6, 2002.
Cardellini, F.: New experimental activity at ENEA INMRI radon laboratory, WORKSHOP: The second radon-in-field international intercomparison for passive measurement devices: dwellings and workplaces, Milano, Italy, https://www.airp-asso.it/wp-content/uploads/convegni/2017_interconfronto/day2/7 Francesco Cardellini.pdf (last access: 2 March 2022), 2017.
Cardellini, F., De Felice, P., and Pagliari, M.: Determination of blank indication of active radon monitors, Appl. Radiat. Isot. 81, 242–247, 2013.
Chambers, S. D., Preunkert, S., Weller, R., Hong, S.-B., Humphries, R. S., Tositti, L., Angot, H., Legrand, M., Williams, A. G., Griffiths, A. D., Crawford, J., Simmons, J., Choi, T. J., Krummel, P. B., Molloy, S., Loh, Z., Galbally, I., Wilson, S., Magand, O., Sprovieri, F., Pirrone, N., and Dommergue, A.: Characterizing Atmospheric Transport Pathways to Antarctica and the Remote Southern Ocean Using Radon-222, Front. Earth Sci., 6, https://doi.org/10.3389/feart.2018.00190, 2018.
Cinelli, G., Tollefsen, T., Bossew, P., Gruber, V., Bogucarskis, K., De Felice, L., and De Cort, M.: Digital version of the European Atlas of natural radiation, J. Environ. Radioactiv., 196, 240–252, https://doi.org/10.1016/j.jenvrad.2018.02.008, 2019.
Dörr, H., Kromer, B., Levin, I., Münnich, K. O., and Volpp, H.-J.: CO2 and radon 222 as tracers for atmospheric transport, J. Geophys. Res.-Oceans, 88, 1309–1313, https://doi.org/10.1029/JC088iC02p01309, 1983.
Dubois, G.: An overview of radon surveys in Europe, Office for Official Publication of the European Communities, Luxembourg, 172 pp., EUR 21892 EN, ISBN 92-79-01066-2, 2005.
DURRIDGE Company Inc.: RAD 7 Electronic Radon Detector User Manual, RAD7 Manual, http://durridge.com (last access: 2 March 2022), 2021.
Friedmann, H.: Final results of the Austrian radon project, Health Phys., 89, 339–348, https://doi.org/10.1097/01.HP.0000167228.18113.27, 2005.
Fialova, E., Otahal, P., Vosahlik, J., and Mazanova, M.: Equipment for Testing Measuring Devices at a Low-Level Radon Activity Concentration, Int. J. Environ. Res. Public Health, 17, 1904, https://doi.org/10.3390/ijerph17061904, 2020.
Griffiths, A. D., Chambers, S. D., Williams, A. G., and Werczynski, S.: Increasing the accuracy and temporal resolution of two-filter radon–222 measurements by correcting for the instrument response, Atmos. Meas. Tech., 9, 2689–2707, https://doi.org/10.5194/amt-9-2689-2016, 2016.
Grossi, C., Arnold, D., Adame, J. A., López-Coto, I., Bolívar, J. P., de la Morena, B. A., and Vargas, A.: Atmospheric 222Rn concentration and source term at El Arenosillo 100 m meteorological tower in southwest Spain, Radiat. Meas., 47, 149–162, https://doi.org/10.1016/j.radmeas.2011.11.006, 2012.
Grossi, C., Vogel, F. R., Morgui, J. A., Curcoll, R., Àgueda, A., Batet, O., Nofuentes, M., Occhipinti, P., Vargas, A., and Rodó, X.: First estimation of CH4 fluxes using the 222Rn tracer method over the central Iberian Peninsula, in Air Pollution XXII, WIT Trans. Ecol. Environ., 183, 233–245, https://doi.org/10.2495/AIR140201, 2014.
Grossi, C., Vogel, F. R., Curcoll, R., Àgueda, A., Vargas, A., Rodó, X., and Morguí, J.-A.: Study of the daily and seasonal atmospheric CH4 mixing ratio variability in a rural Spanish region using 222Rn tracer, Atmos. Chem. Phys., 18, 5847–5860, https://doi.org/10.5194/acp-18-5847-2018, 2018.
Grossi, C., Chambers, S. D., Llido, O., Vogel, F. R., Kazan, V., Capuana, A., Werczynski, S., Curcoll, R., Delmotte, M., Vargas, A., Morguí, J.-A., Levin, I., and Ramonet, M.: Intercomparison study of atmospheric 222Rn and 222Rn progeny monitors, Atmos. Meas. Tech., 13, 2241–2255, https://doi.org/10.5194/amt-13-2241-2020, 2020.
Karstens, U., Schwingshackl, C., Schmithüsen, D., and Levin, I.: A process-based 222radon flux map for Europe and its comparison to long-term observations, Atmos. Chem. Phys., 15, 12845–12865, https://doi.org/10.5194/acp-15-12845-2015, 2015.
Kemski, J., Siehl, A., Stegemann, R., and Valdivia-Manchego, M.: Mapping the geogenic radon potential in Germany, Sci. Total Environ., 272, 217–230, https://doi.org/10.1016/S0048-9697(01)00696-9, 2001.
Khanbabaee, B., Röttger, A., Behrens, R., Röttger, S., Feige, S., Hupe, O., Zutz, H., Toroi, P., Leonard, P., de la Fuente Rosales, L., Burgess, P., Gressier, V., Gutiérrez Villanueva, J.-L., Cruz Suárez, R., and Arnold, D.: Support for a European Metrology Network on Reliable Radiation Protection: Gaps in Radiation Protection and Related Metrology, RAD Conference Proceedings, 5, 21–27, https://doi.org/10.21175/RadProc.2021.04, 2021.
Koffi, E. N., Bergamaschi, P., Karstens, U., Krol, M., Segers, A., Schmidt, M., Levin, I., Vermeulen, A. T., Fisher, R. E., Kazan, V., Klein Baltink, H., Lowry, D., Manca, G., Meijer, H. A. J., Moncrieff, J., Pal, S., Ramonet, M., Scheeren, H. A., and Williams, A. G.: Evaluation of the boundary layer dynamics of the TM5 model over Europe, Geosci. Model Dev., 9, 3137–3160, https://doi.org/10.5194/gmd-9-3137-2016, 2016.
Levin, I., Born, M., Cuntz, M., Langendörfer, U., Mantsch, S., Naegler, T., Schmidt, M., Varlagin, A., Verclas, S., and Wagenbach, D.: Observations of atmospheric variability and soil exhalation rate of Radon-222 at a Russian forest site: Technical approach and deployment for boundary layer studies, Tellus B, 54, 462–475, 2002.
Levin, I., Hammer, S., Eichelmann, E., and Vogel, F.R.: Verification of greenhouse gas emission reductions: the prospect of atmospheric monitoring in the polluted areas, Philos. T. Roy. Soc. A, 369, 1906–1924, https://doi.org/10.1098/rsta.2010.0249, 2011.
Levin, I., Karstens, U., Hammer, S., DellaColetta, J., Maier, F., and Gachkivskyi, M.: Limitations of the radon tracer method (RTM) to estimate regional greenhouse gas (GHG) emissions – a case study for methane in Heidelberg, Atmos. Chem. Phys., 21, 17907–17926, https://doi.org/10.5194/acp-21-17907-2021, 2021.
Linzmaier, D., Röttger, A.: Development of a transfer standard for the measurement of low Rn-222 activity concentration in air, Appl. Radiat. Isot., 87, 306–309, https://doi.org/10.1016/j.apradiso.2013.11.076, 2014.
López-Coto, I., Mas, J. L., and Bolívar, J. P.: A 40-year retrospective European radon flux inventory including climatological variability, Atmos. Environ., 73, 22–33, https://doi.org/10.1016/j.atmosenv.2013.02.043, 2013.
Mazed, D., Ciolini, R., Curzio, G., and Del Gratta, A.: A new active method for continuous radon measurements based on a multiple cell proportional counter, Nucl. Instr. Meth. Res. A, 582, 535–545, 2007.
Mertes, F., Röttger, S., and Röttger, A.: A new primary emanation standard for Radon-222, Appl. Radiat. Isot., 156, 108928, https://doi.org/10.1016/j.apradiso.2019.108928, 2020.
Mertes, F., Röttger, S., and Röttger, A: Approximate Sequential Bayesian Filtering to Estimate Rn-222 Emanation from Ra-226 Sources from Spectra, Proceedings of the Conference Sensor and Measurement Science International, 256–257, ISBN 978-3-9819376-4-0, https://doi.org/10.5162/SMSI2021/D3.3, 2021.
Mi.am Srl/Tecnavia SA – Radon Detectors: RADON MAPPER Technical Specifications, prodotti-dosimetria-radon-mapper EN, http://miam.it (last access: 2 March 2022), 2019.
Neznal, M., Neznal, M., Matolín, M., Barnet, I., and Miksova, J.: The new method for assessing the radon risk of building sites, Czech Geological Survey Special Papers 16, Czech Geological Survey, Prague, 47 pp., https://www.radon-vos.cz/pdf/metodika.pdf (last access: 2 March 2022), 2004.
Olivié, D. J. L., van Velthoven, P. F. J., and Beljaars, A. C. M.: Evaluation of archived and off-line diagnosed vertical diffusion coefficients from ERA-40 with 222Rn simulations, Atmos. Chem. Phys., 4, 2313–2336, https://doi.org/10.5194/acp-4-2313-2004, 2004.
Pierre, S., Cassette, P., Sabot, B., Fréchou, C., Antohe, A., Barna, C., Blahušiak, P., Cardellini, F., Dersch, R., Honig, A., Juget, F., Krivošík, M., Luca, M., Maringer, F. J., Mertes, F., Röttger, S., Sahagia, M., Slučiak, Stietka, M., Szűcs, L., and Teodorescu, C.: International comparison of activity measurements of radon 222, EURAMET Project no. 1475, EURAMET.RI(II)-S8.Rn-222, Metrologia, Volume 58, Number 1A, https://www.bipm.org/documents/20126/48150641/EURAMET.RI(II)-S8.Rn-222.pdf/68464ff6-b2a7-f971-4d93-420086e932f0 (last access: 2 March 2022), 2021.
Polian, G., Lambert, G., Ardouin, B., and Jegou, A.: Long-range transport of continental radon in subantarctic and Antarctic areas, Tellus, 388, 178–189, 1986.
Radulescu, I., Calin, M. R., Luca, A., Röttger, A., Grossi, C., Done, L., and Ioan, M. R.: Inter-comparison of commercial continuous radon monitors responses, NIM-A, 1021, 165927, https://doi.org/10.1016/j.nima.2021.165927, 2022.
Roessler, F.: AlphaGUARD DF User Manual EN Rev.E, Bertin-Instruments, https://www.bertin-instruments.com/wp-content/uploads/2016/09/Brochure_AlphaGUARD_2022_web.pdf (last access: 2 March 2022), 2020.
Röttger, A. and Honig, A.: Recent developments in radon metrology: new aspects in the calibration of radon, thoron and progeny devices, Radiat. Prot. Dosim., 145, 260–266, https://doi.org/10.1093/rpd/ncr047, 2011.
Röttger, A. and Kessler, P.: Uncertainties and characteristic limits of counting and spectrometric dosimetry systems, J. Environ. Radioact., 205–206, 48–54, https://doi.org/10.1016/j.jenvrad.2019.04.012, 2019.
Röttger, A., Veres, A., Sochor, V., Pinto, M., Derlacinski, M., Ioan, M.-R., Sabeta, A., Bernat, R., Adam-Guillermin, C., Gracia Alves, J. H., Glavič-Cindro, D., Bell, S., Wens, B., Persson, L., Živanović, M., and Nylund, R.: Metrology for radiation protection: a new European network in the foundation phase, Adv. Geosci., 57, 1–7, https://doi.org/10.5194/adgeo-57-1-2021, 2021.
SARAD GmbH: RTM2200 Info Sheet, Technical Data, EN 013, https://www.sarad.de/cms/media/docs/handbuch/Manual_RTM-RPM22xx_EQF32xx_A2M4000_EN_24-11-16.pdf, last access: 2 March 2022.
Sangiorgi, M., Hernández-Ceballos, M. A., Jackson, K., Cinelli, G., Bogucarskis, K., De Felice, L., Patrascu, A., and De Cort, M.: The European Radiological Data Exchange Platform (EURDEP): 25 years of monitoring data exchange, Earth Syst. Sci. Data, 12, 109–118, https://doi.org/10.5194/essd-12-109-2020, 2020.
Schmithüsen, D., Chambers, S., Fischer, B., Gilge, S., Hatakka, J., Kazan, V., Neubert, R., Paatero, J., Ramonet, M., Schlosser, C., Schmid, S., Vermeulen, A., and Levin, I.: A European-wide 222radon and 222radon progeny comparison study, Atmos. Meas. Tech., 10, 1299–1312, https://doi.org/10.5194/amt-10-1299-2017, 2017.
Southern Scientific: AB7 – Radon Monitor, Specifications, © 2022 LabLogic Systems Ltd, https://www.southernscientific.co.uk/products-by-manufacturer/pylon/ab6?source=1581#specifications, last access: 2 March 2022.
Stohl, A., Hittenberger, M., and Wotawa, G.: Validation of the Lagrangian particle dispersion model FLEXPART against large scale tracer experiment data Atmos. Environ., 32, 4245–4264, https://doi.org/10.1016/s1352-2310(98)00184-8, 1998.
Streil, T, Oeser, V., and Sabol, J.: RTM 2200 Radon/Thoron monitor – ”System in a box” for complex sampling procedures and multi parameter analysis, PowerPoint-Präsentation, http://elradon.com (last access: 2 March 2022), 2011.
Szegvary, T., Conen, F., and Ciais, P.: European 222Rn inventory for applied atmospheric studies, Atmos. Environ., 43, 1536–1539, https://doi.org/10.1016/j.atmosenv.2008.11.025, 2009.
Tesla: Radon Monitor RADIM 3AT Technical Specifications & Operation Manual, v1-2018, navod radim en, http://tesla.cz (last access: 2 March 2022), 2018.
Tollefsen, T., De Cort, M., Cinelli, G., Gruber, V., and Bossew, P.: European atlas of natural radiation, Publication Office of the European Union, Luxembourg, https://publications.jrc.ec.europa.eu/repository/handle/JRC106967 (last access: 2 March 2022), 2019.
Van Der Laan, S., Karstens, U., Neubert, R. E. M., Van Der Laan-Luijkx, I., and Meijer, H. A. J.: Observation-based estimates of fossil fuel-derived CO2 emissions in the Netherlands using 14C, CO and 222Radon, Tellus B, 62, 389–402, https://doi.org/10.1111/j.1600-0889.2010.00493.x, 2010.
Vargas, A., Arnold, D., Adame, J. A., Grossi, C., Hernández-Ceballos, M. A., and Bolívar, J. P.: Analysis of the vertical radon structure at the spanish “El arenosillo” tower station, J. Environ. Radioact., 139, 1–17, https://doi.org/10.1016/j.jenvrad.2014.09.018, 2015.
Vogel, F. R., Ishizawa, M., Chan, E., Chan, D., Hammer, S., Levin, I., and Worthy, D. E. J.: Regional non-CO2 greenhouse gas fluxes inferred from atmospheric measurements in Ontario, Canada, J. Integr. Environ. Sci., 9, 41–55, https://doi.org/10.1080/1943815X.2012.691884, 2012.
Wada, A., Matsueda, H., Murayama, S., Taguchi, S., Hirao, S., Yamazawa, H., Moriizumi, J., Tsuboi, K., Niwa, Y., and Sawa, Y.: Quantification of emission estimates of CO2, CH4 and CO for East Asia derived from atmospheric radon-222 measurements over the western North Pacific, Tellus B, 65, 18037, https://doi.org/10.3402/tellusb.v65i0.18037, 2013.
Whittlestone, S. and Zahorowski, W.: Baseline radon detectors for shipboard use: Development and deployment in the First Aerosol Characterization Experiment (ACE 1), J. Geophys. Res., 103, 16743–16751, https://doi.org/10.1029/98JD00687, 1998.
This project 19ENV01 traceRadon has received funding from the EMPIR programme co-financed by the Participating States and from the European Union's Horizon 2020 research and innovation programme. 19ENV01 traceRadon denotes the EMPIR project reference.
- Abstract
- Introduction
- Project plan of traceRadon
- Motivation for the need of traceability
- The need for a protocol for the application of the Radon Tracer Method (RTM)
- Radon in Radiation Protection
- New activity standards
- Radon monitors – An overview
- Outlook
- Data availability
- Author contributions
- Competing interests
- Disclaimer
- Special issue statement
- Acknowledgements
- Financial support
- Review statement
- References
- Abstract
- Introduction
- Project plan of traceRadon
- Motivation for the need of traceability
- The need for a protocol for the application of the Radon Tracer Method (RTM)
- Radon in Radiation Protection
- New activity standards
- Radon monitors – An overview
- Outlook
- Data availability
- Author contributions
- Competing interests
- Disclaimer
- Special issue statement
- Acknowledgements
- Financial support
- Review statement
- References