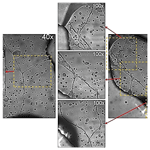
Challenges in experimental investigations of Paracoccus denitrificans activities in salt-bearing porous media
Selen Ezgi Celik
Alejandra Reyes Amezaga
Sebastiaan Godts
Laurenz Schröer
Amir Raoof
Veerle Cnudde
Salt weathering, driven by the crystallization of saline solutions within sedimentary rocks, leads to significant material degradation. Key factors influencing this process include salt type, concentration, moisture levels, temperature fluctuations, and pore structure. Environmental conditions and microbial activity further impact weathering, either mitigating or exacerbating its effects. Microorganisms contribute to biological weathering but may also enhance rock properties through biofilm formation or biocementation. Laboratory techniques such as rock testing and micromodel experiments face challenges in replicating complex interactions between microorganisms and salt-bearing porous materials. In this study, we investigated the activity of Paracoccus denitrificans in saline solutions through experiments conducted on Petri dishes, glass slides and micromodels. Key challenges included replicating controlled conditions, managing contamination, and maintaining strict environmental controls. The results highlighted significant challenges in interpreting bacterial activity within salt-bearing systems, particularly due to contamination risks and difficulties in maintaining precise experimental conditions. Additionally, experimental setups, such as fluid actuation using laser light beams, were found to introduce further complexities. This research provided recommendations and emphasized the critical need for refined methodologies to enhance accuracy and reliability in future studies.
- Article
(1185 KB) - Full-text XML
- BibTeX
- EndNote
Weathering is the natural phenomenon by which rocks are broken down and altered by physical, chemical, and biological processes (Smith et al., 2008). This phenomenon significantly impacts the built infrastructure, leading to the gradual disintegration and decomposition of materials. Salt weathering is a common weathering process, which involves saline solutions moving through porous materials with repeated crystallization and dissolution often leading to material damage (Desarnaud et al., 2013; Derluyn et al., 2014). This phenomenon can be observed both in coastal areas due to high salt concentrations from seawater and also in various structures within the built environment, where cycles of wetting and drying occur (Godts et al., 2023).
Key factors influencing salt weathering include the type and concentration of salts, moisture content, temperature and air humidity fluctuations, and material properties such as strength and pore structure (Grossi et al., 2011; Shen et al., 2024). In this process, the distribution and movement of moisture inside a porous system plays a crucial role. Moisture can infiltrate the rock pore space through mechanisms like capillary action and water vapor transport, supplying dissolved salt or transporting and depositing them in pores and cracks (Oguchi and Yu, 2021). As brine evaporates, salt crystals form and may exert pressure on the surrounding material (Flatt et al., 2017; Steiger, 2005). Salt crystallization is further affected by environmental factors such as fluctuations in relative humidity and temperature, which impact the drying kinetics (Desarnaud et al., 2015).
Besides the presence of salts, microbial organisms are also present on stone. They are generally known to adversely alter rock surfaces and cause biodeterioration (Schröer et al., 2021). However, some studies suggest that microorganisms can positively affect rock properties by forming biofilms and producing biocement (Jroundi et al., 2010; Rodriguez-Navarro et al., 2012; Ranalli and Zanardini, 2021; De Muynck et al., 2011). This complexity complicates the development of methods to introduce beneficial microorganisms for stone bioremediation, bio-consolidation, or bio-protection. A key challenge lies in ensuring the adhesion and survival of inoculated microbial organisms on stone surfaces. Limited nutrients and harsh environmental conditions, such as temperature and humidity fluctuations, combined with exposure to pollutants can compromise microbial viability.
Equally important is the risk of undesirable interactions between inoculated microorganisms and stone material and minerals and thereby potentially altering salt crystallization. Microorganisms may mitigate or worsen weathering, making it crucial to understand their impact on stone weathering/conservation (Goudie and Viles, 1997). Despite numerous publications on salt and biological weathering, their interaction and, the effects of different salt (mixture) types, concentration, and ionic strength on microbially mediated reactions within porous media are not well understood. High salt environments can cause osmotic stress in bacterial cells, leading to dehydration, disruption of cellular functions, and even cell death (Wood, 2015). Elevated salt levels create hypertonic conditions causing plasmolysis, which disrupts microbial growth and function. Furthermore, ions like Na+ and Cl− can be toxic to bacteria at high concentrations, inhibiting enzyme activities and nutrient uptake. High salinity can affect nutrient availability and can alter bacterial metabolism, complicating cultivation and experimental reproducibility.
To understand the salt-bacteria interactions in porous systems during evaporation, one efficient way is to look at their behavior in microfluidic devices subject to varying environmental conditions (Zhou et al., 2019). Micromodels mimic partially the pore structure of rocks and due to their transparency and thin design enable the visualization of fluid transport at the microscale, making them popular in biomedical and microbiology research (Sackmann et al., 2014). Despite their advantages, measurements conducted using micromodels present several challenges (Karadimitriou and Hassanizadeh, 2012). For instance, the use of light and laser beams is a fundamental component of microfluidic studies. However, some studies have highlighted the potential effects of localized laser heating, such as the formation of bubbles within the liquid inside micromodels (Hung and Huang, 2013). When investigating microbial activity within microfluidic devices as well as the dynamic interactions between microorganisms and their surrounding environments, it is crucial to thoroughly address the associated challenges to ensure accurate and reliable insights. These challenges include the precise replication of natural microenvironments, the potential artifacts introduced by experimental setups, and the limitations of current imaging and analytical techniques.
Investigating the interplay between bacterial activity and salt crystallization in porous media, such as micromodels, requires addressing several key challenges. These include assessing bacterial influence on salt crystallization. This involves exploring how bacterial growth and biofilm formation are influenced by varying salt concentrations and assessing their potential role in shaping salt crystallization patterns. Since bacteria require an optimal growth medium to form biofilms, the introduction of such a medium into a porous structure may inadvertently facilitate salt crystallization by altering local chemical and physical conditions. Conversely, addressing the potential challenges of fostering bacterial growth in salt-saturated porous media is essential. In this context, a critical question arises regarding bacterial activities and interactions in saline environments, specifically concerning the survival and resilience of bacteria in the presence of specific ions and under high-salinity conditions.
Biological contamination can obscure the extent of the engineered bacterial colonization inside porous structures, complicating result interpretation. These challenges add to the complexity of microbial research in saline conditions within rocks. Building on the previously mentioned facts, it is essential to identify potential artefacts and challenges when using specific bacteria in research studies across different scales and techniques. This includes understanding how bacteria selection and experimental setup can impact results and recognizing sources of error or distortion. Addressing these factors will optimize research methods and improve result reliability.
A critical challenge in biological experimentation within micromodels is contamination control. Maintaining sterility across equipment, media, and experimental environments is critical but inherently difficult. It requires rigorous protocols and careful handling to prevent microbial contamination. Implementing effective contamination control measures is essential to ensure reliable and reproducible results in microfluidic-based microbial studies. Sterilization procedures like autoclaving, chemical disinfectants like isopropyl alcohol or ethanol, and even ultraviolet (UV) irradiation might be necessary but may add some complexity (Bharti et al., 2022; Harrigan and Mccance, 2014). Maintaining sterility in micromodel equipment is challenging due to limited disinfectant access to tiny pores, human error, handling issues, and airborne microorganisms. Cross-contamination in multi-experiments requires strict protocols and early detection of contamination is time-consuming. Failure to maintain sterility can lead to unwanted microbial growth, compromising research outcomes.
In this study, we explored the interactions between the bacterial activity of Paracoccus denitrificans, a specific denitrifying bacterium, under varying concentrations of saline solutions through experiments conducted on Petri dishes, glass slides and micromodels and highlight the associated experimental challenges and potential mitigation. Special attention was devoted to examining evaporation from bio-treated saturated porous samples to evaluate the influence of bacterial presence on the evaporation process, salt crystallization, and biofilm growth. We selected P. denitrificans for its potential application in soil improvement (Rebata-Landa and Santamarina, 2012) and building stone conservation (Schröer, 2017).
The experiments conducted in this study utilized a mixture of P. denitrificans, mineral medium, and either water or various salt solutions. Two types of experiments were designed to evaluate bacterial interactions with different salts: aqueous solution experiments in (1) test tubes, and (2) micromodels.
2.1 Aqueous solution experiments in test tubes
To test the viability of P. denitrificans in high-concentration salt solutions, a series of experiments were conducted using the following mixtures: the growth medium (Table 1) described by Erşan et al. (2015), the same medium without salts, the medium with only salts, and salts prepared according to RILEM standards (5 wt % and 10 wt % NaCl and Na2SO4). The mixtures were inoculated with P. denitrificans strains grown in Lysogeny Broth (LB) medium and incubated at 25 °C for 21 d. Samples were collected on days 1, 3, 7, 14, and 21 for subsequent plating tests. The plating method was employed to assess bacterial viability under the specified experimental conditions.
2.2 Aqueous solution experiments in micromodels
Figure 1a provides a snapshot of a saturated micromodel used in the study, while the procedure for the evaporation experiment conducted in the micromodel is detailed in Fig. 1b. The micromodel was fabricated from Polydimethylsiloxane (PDMS), a transparent silicon polymer, mimicking a slice of a homogeneous porous stone with a porosity of 40 % and a thickness of 150 µm. Prior to conducting the experiment, bacterial cultures grown in LB were centrifuged and subsequently mixed with a methanol-mineral medium, following the method described by Erşan et al. (2015), to prepare the bacterial solution. Initially, we saturated all micromodels with deionized (DI) water or different salt solutions at relatively high concentrations, including sodium chloride (21.2 wt %), sodium sulphate (15.0 wt %), and a mixture of these two salts (NaCl 3.9 wt %; Na2SO4 9.6 wt %). Following this, 210 µL of the bacterial solution was injected with a 1 µL min−1 rate in the open channel of the micromodel interface (Fig. 1a) and then incubated the micromodels for 3 to 5 d. Afterward, they were partially dried by introducing air at varying relative humidity levels, while maintaining ambient temperatures, at a flow rate of 100 cm3 STP min−1 across the model's interface. Finally, we examined the micromodels using Confocal Laser Scanning Microscope (CLSM) (Nikon ECLIPSE Ti) at magnifications ranging from 40x to 100x.
3.1 Aqueous solution experiments in test tubes
3.1.1 Activity of P. denitrificans under high-salinity conditions
Preliminary studies in test tubes conducted in this research indicated that while P. denitrificans can survive in higher concentrations of Na2SO4 up to 10 wt %, the tolerance threshold for NaCl is lower (5 wt %). The literature has limited information regarding the salt tolerance of this bacteria. However, based on the work of Nokhal and Schlegel (1983), it has been noted they can withstand salt solutions up to 7 wt % NaCl concentrations. It is known that most cultural heritage buildings have salt within the pores (Steiger et al., 2014), and determining the salt concentrations and ion compositions that P. denitrificans can tolerate is crucial for its potential application in the field of conservation. On the other hand, P. denitrificans needs a certain mineral medium for optimal growth. However, these media typically contain low amounts of salts, usually below 5 wt % (Al-Salloum et al., 2017). One of the main challenges we faced during the test tube experiments, was contamination as observed in the plating tests (Fig. 2).
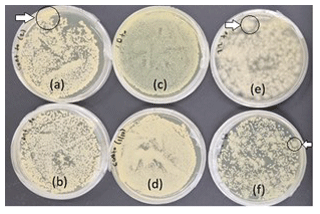
Figure 2Plating test results from the salt tolerance experiment, with white arrows indicating contaminations: (a, b, d) Pure P. denitrificans samples suspended in water. Contamination is observed as a small spot in (a). (c) P. denitrificans grown with only salts in Ersan's medium (Erşan et al., 2015). (e) P. denitrificans in full Ersan's medium, with contamination almost fully covering the plate. (f) P. denitrificans in Ersan's medium without salts, with partial contamination present.
3.1.2 Salt morphological changes by bacteria during drying
Another observed outcome of salt and bacteria interactions is the morphological changes in salt crystallization in the presence of bacteria. For example, in Fig. 3, slide images of 5 wt % and 10 wt % NaCl and Na2SO4 salt solutions are shown. As evident from these images, the crystallization patterns differ significantly in the presence of bacteria (either dead or alive). This suggests that when salts are present in stones, post-application drying can result in varying forms of deposition.
3.2 Aqueous solution experiments in micromodels
3.2.1 Fluid actuation by laser/light beams
Figure 4 illustrates an example of the evolution of the interface between bacteria-containing liquid and air-dried regions during imaging by CLSM. Over time, the interface distorts due to localized heating. We found that the high-intensity beams negatively (i.e., destructively) affected the liquid-gas interface. In other words, the interface temporally evolved during the CLSM measurements and lost its original morphology. This heating can alter surface tension and contact angles, leading to changes in fluid distribution and potentially inaccurate experimental results. Additionally, the heat may accelerate evaporation, further distorting observations and affecting the interpretation of transport processes.
3.2.2 Bacteria-salt interactions in microfluidic experiments
Figure 5 illustrates micromodel images after the drying test, where the model was saturated with an NaCl-Na2SO4 brine mixture
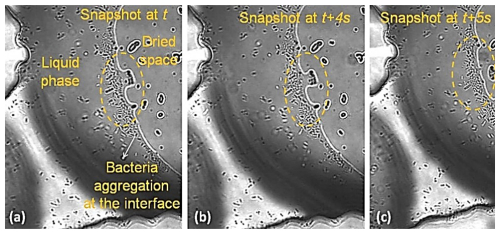
Figure 4An example of the temporal evolution of the liquid-gas interface between bacteria-containing liquid and air-dried regions during CLSM imaging, influenced by laser beam effects; A snapshot of a liquid-air interface at time (a) t, (b) t+4 s, and (c) t+5 s.
prior to the injection of a bacteria-containing liquid. Here, we observed a biological network inside the model. Further identification is needed to determine whether this network originated from P. denitrificans activity or from other microbial organisms, as it may alter salt crystallization mechanisms and kinetics. This becomes more important when the saline solutions inside the model undergo an evaporation process, increasing the possibility of crystallization with different morphologies and structures. The potential occurrence of ring effects (Yanni et al., 2017), salt crystallization and biomass aggregation/biofilm creates a very complex situation that necessitates the use of additional techniques, such as scanning electron microscopy (SEM), to accurately distinguish these features.
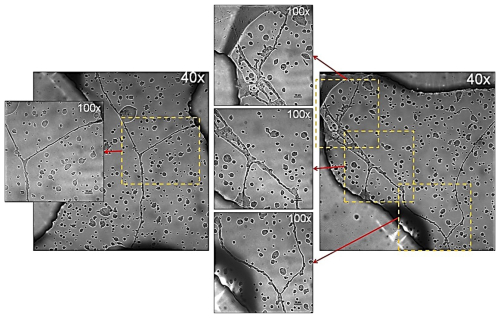
Figure 5Examples of CLSM images after the drying experiment for models saturated with NaCl-Na2SO4+ bacteria-containing liquid. Evidence of hyphae-like structures or potential biofilms are visible in the partially dried pore space. Further analysis is necessary to confirm the composition of these structures.
3.2.3 Maintaining experimental validity in micromodel studies
Contamination control is crucial for reliable micromodel microbial experiments, as even minor contamination can misrepresent bacterial interactions with salt solutions. We found that unwanted microorganisms, such as airborne microbes or potentially fungi, could introduce variability and interfere with the experimental outcomes. Strict sterility is therefore essential, including the use of sterilized tools, proper sealing, laminar flow hoods, and cleanrooms to prevent exposure to airborne microorganisms. Additionally, specific contamination protocols should be tailored to the microorganisms under study to maintain the integrity of the experimental setup.
The use of microorganisms in experimental studies usually requires replicates to confirm their findings. In this study, multiple iterations were conducted to ensure the reproducibility of observations and to minimize the influence of random variation or experimental errors. Achieving consistent and reproducible results in microbiological systems, particularly in micromodel studies, proved challenging due to inherent variations in bacterial growth and behavior. Maintaining optimal conditions for bacterial activity, such as temperature, relative humidity, and pH, required precise control and monitoring, as even slight deviations could affect outcomes. One significant observation during our experiments involved the formation of hyphae-like structures within micromodels. However, based on imaging data alone, it remains unclear whether these structures represent biomass aggregations, biofilms, fungal hyphae, or other phenomena. Additional research, including advanced characterization methods such as SEM and various spectroscopy techniques, is required to conclusively identify these structures. Furthermore, contamination emerged as a critical concern, as even minor contamination at this scale could complicate interpretations and undermine the validity of results.
Working with P. denitrificans in saline environments presented unique challenges, including controlling environmental conditions, managing contamination risks, and addressing issues related to environmental chemistry and imaging components, such as laser beam-line effects. These challenges were particularly pronounced during drying experiments involving bacterial-inoculated micromodels and stone materials. Despite these difficulties, our preliminary results demonstrated the feasibility of studying bacterial dynamics in salt-bearing porous media. Future research should focus on distinguishing bacterial-induced products generated by P. denitrificans from those generated due to contamination. A combination of different techniques can provide more relevant data. This will enhance our understanding of microbial behavior in complex environments and improve the accuracy of experimental outcomes.
The data of this study are available from the corresponding author upon reasonable request.
JQ and SEC: Conceptualization, Investigation, Methodology, Visualization, Writing – original draft preparation; ARA: Conceptualization, Writing – review & editing; SG and LS: Writing – review & editing; AR: Resources, Software, Writing – review & editing; VC: Conceptualization, Funding acquisition, Supervision, Writing – review & editing.
The contact author has declared that none of the authors has any competing interests.
Publisher’s note: Copernicus Publications remains neutral with regard to jurisdictional claims made in the text, published maps, institutional affiliations, or any other geographical representation in this paper. While Copernicus Publications makes every effort to include appropriate place names, the final responsibility lies with the authors.
This article is part of the special issue “European Geosciences Union General Assembly 2024, EGU Division Energy, Resources & Environment (ERE)”. It is a result of the EGU General Assembly 2024, Vienna, Austria, 14–19 April 2024.
The authors express their gratitude to GeoLab at Utrecht University, with special thanks to Mahin Baghery and Qianjing Tang for their assistance in microfluidic experiments. Additionally, they extend their appreciation to UGCT at Ghent University for conducting the micro-CT scanning.
This project has received funding from the Dutch Research Council (NWO) through the BugControl project (project number VI.C.202.074) of the NWO Talent program; the EXCITE network, part of the European Union's Horizon 2020 research and innovation program (grant agreement no. 101005611) and from Ghent University Special Research Fund (BOF-UGent) to support the UGent Core facility UGCT (grant no. BOF.COR.2022.0009).
This paper was edited by Sonja Martens and reviewed by two anonymous referees.
Al-Salloum, Y., Hadi, S., Abbas, H., Almusallam, T., and Moslem, M. A.: Bio-induction and bioremediation of cementitious composites using microbial mineral precipitation – A review, Construct. Build. Materials, 154, 857–876, https://doi.org/10.1016/j.conbuildmat.2017.07.203, 2017.
Bharti, B., Li, H., Ren, Z., Zhu, R., and Zhu, Z.: Recent advances in sterilization and disinfection technology: A review, Chemosphere, 308, 136404, https://doi.org/10.1016/j.chemosphere.2022.136404, 2022.
De Muynck, W., Leuridan, S., Van Loo, D., Verbeken, K., Cnudde, V., De Belie, N., and Verstraete, W.: Influence of Pore Structure on the Effectiveness of a Biogenic Carbonate Surface Treatment for Limestone Conservation, Appl. Environ. Microbiol., 77, 6808–6820, https://doi.org/10.1128/AEM.00219-11, 2011.
Derluyn, H., Dewanckele, J., Boone, M. N., Cnudde, V., Derome, D., and Carmeliet, J.: Crystallization of hydrated and anhydrous salts in porous limestone resolved by synchrotron X-ray microtomography, Nuclear Instrum. Method. Phys. Res. B, 324, 102–112, https://doi.org/10.1016/j.nimb.2013.08.065, 2014.
Desarnaud, J., Bertrand, F., and Shahidzadeh-Bonn, N.: Impact of the Kinetics of Salt Crystallization on Stone Damage During Rewetting/Drying and Humidity Cycling, J. Appl. Mechan., 80, 020911, https://doi.org/10.1115/1.4007924, 2013.
Desarnaud, J., Derluyn, H., Molari, L., de Miranda, S., Cnudde, V., and Shahidzadeh, N.: Drying of salt contaminated porous media: Effect of primary and secondary nucleation, J. Appl. Phys., 118, 114901, https://doi.org/10.1063/1.4930292, 2015.
Erşan, Y. Ç., Belie, N. D., and Boon, N.: Microbially induced CaCO3 precipitation through denitrification: An optimization study in minimal nutrient environment, Biochem. Eng. J., 101, 108–118, https://doi.org/10.1016/j.bej.2015.05.006, 2015.
Flatt, R., Aly Mohamed, N., Caruso, F., Derluyn, H., Desarnaud, J., Lubelli, B., Espinosa Marzal, R. M., Pel, L., Rodriguez-Navarro, C., Scherer, G. W., Shahidzadeh, N., and Steiger, M.: Predicting salt damage in practice: A theoretical insight into laboratory tests, RILEM Technical Letters, 2, 108–118, https://doi.org/10.21809/rilemtechlett.2017.41, 2017.
Godts, S., Orr, S. A., Steiger, M., Stahlbuhk, A., De Kock, T., Desarnaud, J., De Clercq, H., and Cnudde, V.: Salt mixtures in stone weathering, Sci. Rep., 13, 13306, https://doi.org/10.1038/s41598-023-40590-y, 2023.
Goudie, A. S. and Viles, H. A.: Salt Weathering Hazards, Wiley, ISBN 978-0-471-95842-0, 256 pp., 1997.
Grossi, C. M., Brimblecombe, P., Menéndez, B., Benavente, D., Harris, I., and Déqué, M.: Climatology of salt transitions and implications for stone weathering, Sci. Total Environ., 409, 2577–2585, https://doi.org/10.1016/j.scitotenv.2011.03.029, 2011.
Harrigan, W. F. and McCance, M. E.: Laboratory Methods in Microbiology, Elsevier Science, ISBN 9781483255309, 374 pp., 2014.
Hung, M.-S. and Huang, Y.-T.: Laser-induced heating for cell release and cellular DNA denaturation in a microfluidics, BioChip J., 7, 319–324, https://doi.org/10.1007/s13206-013-7402-6, 2013.
Jroundi, F., Fernández-Vivas, A., Rodriguez-Navarro, C., Bedmar, E. J., and González-Muñoz, M. T.: Bioconservation of Deteriorated Monumental Calcarenite Stone and Identification of Bacteria with Carbonatogenic Activity, Micro. Ecol., 60, 39–54, https://doi.org/10.1007/s00248-010-9665-y, 2010.
Karadimitriou, N. K. and Hassanizadeh, S. M.: A Review of Micromodels and Their Use in Two-Phase Flow Studies, Vadose Zone J., 11, https://doi.org/10.2136/vzj2011.0072, 2012.
Nokhal, T.-H. and Schlegel, H. G.: Taxonomic Study of Paracoccus denitrificans, Int. J. Syst. Evolut. Microbiol., 33, 26–37, https://doi.org/10.1099/00207713-33-1-26, 1983.
Oguchi, C. T. and Yu, S.: A review of theoretical salt weathering studies for stone heritage, Prog. Earth Plane. Sci., 8, 32, https://doi.org/10.1186/s40645-021-00414-x, 2021.
Ranalli, G. and Zanardini, E.: The Role of Microorganisms in the Removal of Nitrates and Sulfates on Artistic Stoneworks, in: Microorganisms in the Deterioration and Preservation of Cultural Heritage, edited by: Joseph, E., Springer International Publishing, Cham, 263–279, https://doi.org/10.1007/978-3-030-69411-1_12, 2021.
Rebata-Landa, V. and Santamarina, J. C.: Mechanical Effects of Biogenic Nitrogen Gas Bubbles in Soils, J. Geotech. Geoenviron. Eng., 138, 128–137, https://doi.org/10.1061/(ASCE)GT.1943-5606.0000571, 2012.
Rodriguez-Navarro, C., Jroundi, F., Schiro, M., Ruiz-Agudo, E., and González-Muñoz María, T.: Influence of Substrate Mineralogy on Bacterial Mineralization of Calcium Carbonate: Implications for Stone Conservation, Appl. Environ. Microbiol., 78, 4017–4029, https://doi.org/10.1128/AEM.07044-11, 2012.
Sackmann, E. K., Fulton, A. L., and Beebe, D. J.: The present and future role of microfluidics in biomedical research, Nature, 507, 181–189, https://doi.org/10.1038/nature13118, 2014.
Schröer, L.: X-ray CT-technology revealing the effects of denitrifying bacteria on porous limestone, MSc Thesis, Ghent University, Ghent, Belgium, 2017.
Schröer, L., Boon, N., De Kock, T., and Cnudde, V.: The capabilities of bacteria and archaea to alter natural building stones – A review, Int. Biodeterior. Biodegrad., 165, 105329, https://doi.org/10.1016/j.ibiod.2021.105329, 2021.
Shen, Y., Liang, C., Steiger, M., Cao, Z., and Sun, M.: Quantitative analysis on the impact factors of salt weathering for sandstone grottoes along Silk Road, China, J. Cultural Herit., 67, 522–533, https://doi.org/10.1016/j.culher.2024.04.011, 2024.
Smith, B. J., Gomez-Heras, M., and McCabe, S.: Understanding the decay of stone-built cultural heritage, Prog. Phys. Geogr., 32, 439–461, https://doi.org/10.1177/0309133308098119, 2008.
Steiger, M.: Crystal growth in porous materials – II: Influence of crystal size on the crystallization pressure, J. Cryst. Growth, 282, 470–481, https://doi.org/10.1016/j.jcrysgro.2005.05.008, 2005.
Steiger, M., Charola, A. E., and Sterflinger, K.: Weathering and Deterioration, in: Stone in Architecture: Properties, Durability, edited by: Siegesmund, S. and Snethlage, R., Springer Berlin Heidelberg, Berlin, Heidelberg, 225–316, https://doi.org/10.1007/978-3-642-45155-3_4, 2014.
Wood, J. M.: Bacterial responses to osmotic challenges, J/ General Physiol/, 145, 381–388, https://doi.org/10.1085/jgp.201411296, 2015.
Yanni, D., Kalziqi, A., Thomas, J., Ng, S. L., Vivek, S., Ratcliff, W. C., Hammer, B. K., and Yunker, P. J.: Life in the coffee-ring: how evaporation-driven density gradients dictate the outcome of inter-bacterial competition, arXiv: Soft Condensed Matter, arXiv 1707.03472, https://doi.org/10.48550/arXiv.1707.03472, 2017.
Zhou, W., Le, J., Chen, Y., Cai, Y., Hong, Z., and Chai, Y.: Recent advances in microfluidic devices for bacteria and fungus research, TrAC Trends. Anal. Chem., 112, 175–195, https://doi.org/10.1016/j.trac.2018.12.024, 2019.