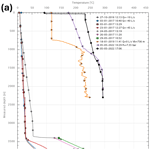
Chemical composition of the discharge fluid from IDDP-2, Reykjanes, Iceland
Iwona Galeczka
Finnbogi Óskarsson
Kiflom Gebrehiwot Mesfin
Jón Einar Jónsson
Well IDDP-2 was drilled through deepening of RN-15 which is one of the geothermal wells producing from the Reykjanes field (Iceland). It was drilled in 2016 and 2017 as part of the Iceland Deep Drilling Project (IDDP), which aim has been to assess the economic viability of supercritical fluids utilization. Well testing, temperature and pressure logging of RN-15/IDDP-2 together with sampling of the discharge fluid was carried out in 2022 to investigate the well production properties and its feeding aquifers. The chemical composition of the discharge samples obtained during the recent RN-15/IDDP-2 flow test in 2022 suggests a slightly higher reservoir temperature of 294 °C compared to 290 °C in 2016. Most of the major non-volatiles are in a low range of the concentrations calculated for the Reykjanes deep reservoir. The RN-15/IDDP-2 feeding aquifer is, however, enriched in gases such as CO2, H2S, N2, and H2 compared to their content before drilling. In general, the temperature, gas content and the discharge composition suggest that part of the fluid entering the well originates at greater depths compared to the depths of the main feed zones in other Reykjanes wells. Additional research is required to evaluate if the discharge of the well has a signature of a fluid that originates at depths corresponding to supercritical conditions of seawater (T > 403 °C, P > 285 bar).
- Article
(4691 KB) - Full-text XML
- BibTeX
- EndNote
The Reykjanes geothermal system is located on the SW-tip of the Reykjanes Peninsula in SW-Iceland (Fig. 1a). Well IDDP-2 at Reykjanes was drilled by Iceland Drilling Ltd. for HS Orka Ltd. in 2016 and 2017 through deepening of one of the production wells, RN-15, from its initial depth of 2507 m to the final slant depth of 4659 m and therefore becoming the deepest well drilled into a high temperature geothermal system (summarized in Friðleifsson et al., 2020). The well was drilled as part of the Iceland Deep Drilling Project (IDDP), which explores the potential of extracting fluids under supercritical conditions from high temperature geothermal fields, thus producing up to ten times more energy than a conventional geothermal well (Friðleifsson and Albertsson, 2000; Friðleifsson et al., 2020). Supercritical conditions are found at the roots of volcanic-hosted geothermal systems and have been encountered during the drilling in the USA, Japan, Italy, Iceland, Mexico, and Kenya (e.g., Reinsch et al., 2017). Although these fluids contain much more heat, they can be difficult to handle. As shown during testing of the well IDDP-1 in Krafla (N-Iceland), the discharge fluid of 440 °C, pressure of 140 bar, caused corrosion and silica dust erosion and deposition (Ármannsson et al., 2014; Elders et al., 2014; Hauksson et al., 2014). Despite similar downhole temperature of 430 °C and pressure of 340 bar estimated at the bottom of RN-15/IDDP-2 supercritical fluid was never discharged due to substantial contribution of shallow feed zones to the overall flow into this well. The logging data collected during drilling, thermal recovery and during well testing suggested three feed zones, at approximate measured depths of 2395, 3400 and 4500 m, however, as estimated originally by Sæther (2020) 90 %–95 % of the discharge fluid would originate from the middle feed zone and the rest from the deepest feed zone. Unfortunately, during the well stimulation, a damaged casing just below 2300 m depth was observed. This depth is close to the depth of the main feed zone of RN-15 before drilling IDDP-2 which had been cased off during the deepening of the well. To this day, this damage hinders any logging below that depth and most likely changes the contribution of various feed zones to the total well discharge. Well simulations showed a relatively large amount of fluid flowing from the deepest zone if the total well discharge is low (Sæther, 2020). Up to date no chemical sample of the fluid from the deepest feed zone has been collected.
In this short contribution, we would like to report the chemical data collected during the most recent RN-15/IDDP-2 step-rate flow test that was carried out on 5 May 2022 after the well continuous discharging for about half a year. Reporting on the current status of the well performance is important for several reasons. First, since this well has been unsuccessful in terms of productivity compared to the other wells in Reykjanes, it has rarely been sampled and flow tested after the drilling was concluded. This highlights the value of the collected data. Second, the blockage due to casing damage made it impossible to log the well at greater depths, therefore the chemical composition of the discharge can help to estimate the contribution of various feeding zones in this well and whether it is financially beneficial to stimulate the well for energy production. Third, the data collected during this study contributes to the general understanding of the Reykjanes geothermal system, especially in the midst of the Reykjanes volcanic unrest (e.g., Sigmundsson et al., 2024). Furthermore, there is a great interest to continue deep drilling into high-temperature geothermal systems in Iceland, therefore the data on the current performance of such deep wells will contribute to the design and planning of risk mitigation for the future IDDP wells.
Reykjanes Peninsula is covered by basalt lavas erupted in post-glacial times (less than 12 500 years ago) along with low-rise hyaloclastite ridges that were formed during the last glacial period (Sæmundsson et al., 2020). The lithology is dominated by pillow basalts, tuffaceous volcanics and sediments, lavas, and basalt intrusions (dikes and sills) that may reach up to 60 % of the successions at deeper levels (Weisenberger et al., 2019). A simple lithological model of the Reykjanes geothermal system is shown in Fig. 1b. Hydrothermal alteration increases from zeolite grade to amphibolite grade with depth (Zierenberg et al., 2021). The secondary minerals that are observed in the subsurface based on drill cuttings from RN-15/IDDP-2 and other wells in the area are epidote, quartz and pyrite/chalcopyrite, garnet. actinolite, and sulfides (pyrite and chalcopyrite; Weisenberger et al., 2019).
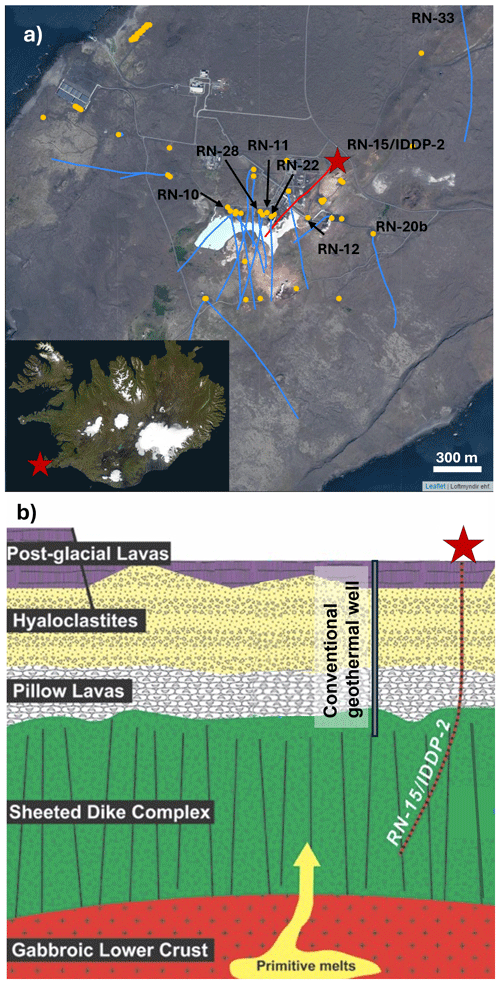
Figure 1The location of the RN-15/IDDP-2 well (red star) and the other geothermal wells drilled in the Reykjanes field (yellow dots) is shown in (a). The trajectories of the wells are given in (a) as red and blue lines. A simplified geological model of the subsurface is shown in (b); modified from Friðleifsson et al. (2017).
At ∼ 4500 m depth, the metabasalt and metagabbro show hydrothermal alteration to amphibole-plagioclase-clinopyroxene-orthopyroxene at temperatures from 700 to > 900 °C, with median temperatures of ∼800 °C (Friðleifsson et al., 2020; Zierenberg et al., 2021).
The geothermal production reservoir of 270–310 °C is at about 800–2300 m depth. The fluid is hydrothermally modified seawater with some addition of magmatic gases. Based on the enthalpy measurements, most of the aquifer is liquid dominated but a few wells have enthalpy higher than that of pure water (Arnórsson, 1978; Freedman et al., 2009; Hardardóttir et al., 2009; Čypaitė et al., 2018; Galeczka and Óskarsson, 2019; Khodayar et al., 2018). Furthermore, the high-temperature conditions of amphibolite facies were confirmed by the temperature and pressure logs which indicate the presence of a supercritical fluid (Friðleifsson et al., 2020).
3.1 Discharge sampling during the flow test
During the flow test the RN-15/IDDP-2 well discharged into a transportable steam separator, which is commonly used for flow testing of geothermal wells (Zarrouk and McLean, 2019). The parameters recorded during the flow test were wellhead pressure (WHP), pressures (P) at various locations in the pipeline towards the separator, lip-pipe P, steam flow rate in the chimney of the separator, liquid flow rate, temperature (T) in the outlet of the separator, and downhole P and T. Further details of the flow testing can be found in Jónsson and Galeczka (2022). The downhole P and T was measured using a Kuster K10 probe that was lowered down through a lubricator attached to the top of the well head. This probe is a memory tool and measures P and T at predetermined time steps. The flow from the well was regulated by a control valve and its opening is given either in cm or as the percentage of full opening. Before the test, the well had been discharging for about half a year at a relatively stable WHP of about 7.8 bar-g and at the valve opening of 22 cm corresponding to 100 % opening. The first discharge sample (liquid, vapor and condensate) during this study was taken at these conditions. Another two samples for analyses of non-condensable gases in vapor were collected at the end of the two latter steps, at 4 cm (18 %) and 1.2 cm (5 %) opening, respectively. The duration of each step was determined by the stabilization of the temperature and pressure when the opening of the valve was changed, and the samples were collected at the end of each step.
Table 1The conditions and the gas content in vapor samples collected during the flow test. Concentrations are given in mg kg−1 of steam. Ps and Ts represents sampling pressure and temperature, respectively.
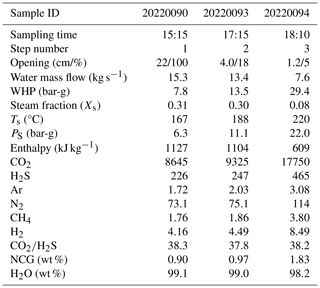
The sampling pressure (Ps) during sample collection was measured at a portable Webre separator, whereas the sampling temperature (Ts) was calculated based on the steam tables (E.T., 2003; Table 1). The simultaneous downhole P and T measurements during the sampling were taken at 2300 m depth, just above the casing damage. The vapor phase was sampled for (1) non-condensable gas content (NCG; Table 1) using evacuated Giggenbach bottles with 40 % NaOH and (2) chosen dissolved components after cooling and condensing the steam at T<20 °C. These components are used as quality check of the phase separation during the sampling. Similar cooling temperature was applied to condense the liquid phase sampled for pH, dissolved solids and isotopic composition. Details on sampling and preservation methods can be found in Arnórsson (2000) and Ólafsson and Ármannsson (2006). Gases in the steam, solutes in condensate and dissolved constituents in the water phase were analysed at the Iceland Geosurvey (ÍSOR) laboratory. The isotope ratios were measured at the Isotech laboratory in USA.
3.2 Deep fluid calculations
The deep fluid composition was calculated based on the chemical composition of the liquid and steam sampled at the surface in step 1 using WATCH computer code version 2.4 (Arnórsson et al., 1982; Bjarnason, 2010). The fluid composition at depth was calculated assuming (1) conservation of enthalpy upon boiling, (2) the fluid was a single-phase liquid in the deep reservoir, and (3) that the measured enthalpy of well discharge represents the total enthalpy of the system according to Eq. (1):
where htotal is the total enthalpy of the system, is the enthalpy of single liquid phase at the reservoir temperature, and hmeasured is the measured well discharge enthalpy. Such wells are referred to as having liquid enthalpy or liquid dominated well discharge. The quartz geothermometer (Fournier and Potter, 1982) was used as a reservoir estimated temperature. This temperature has usually been in a good agreement with the logged down-hole temperatures in Reykjanes wells (e.g., Óskarsson et al., 2015; Galeczka and Óskarsson, 2019).
4.1 Temperature logs
Selected temperature profiles, from during and after drilling of well RN-15/IDDP-2 together with the profiles collected during the flow test performed in this study are depicted in Fig. 2a. The temperature logs collected in 2016–2017 during drilling showed a rapid increase in temperature below approximately 3400 m, when cold water was injected on the wellhead. The temperature logs collected in January 2017 indicate an inflow of hotter fluid through the casing damage at around 2300 m depth as showed by the slight increase of the measured temperature. The inflow, however, at that time was probably limited. During the current test the temperature profile was retrieved only down to about 2300 m depth, as it was not possible to go through the damaged casing. The highest logged temperature in the well was just below 294 °C at 2300 m.
4.2 Fluid composition
The sampling conditions and the gas concentrations in all vapour samples collected during the 2022 flow test are given in Table 1. The results of the chemical analyses for the two-phase sample (20220090) and the calculated deep fluid composition based on this sample are given in Fig. 2b. The deep fluid temperature was calculated to be 293.5 and 244.6 °C using quartz and geothermometers, respectively. The salinity corrected quartz temperature of the deep fluid was calculated to be 291 °C and the estimated steam fraction was 0.31.
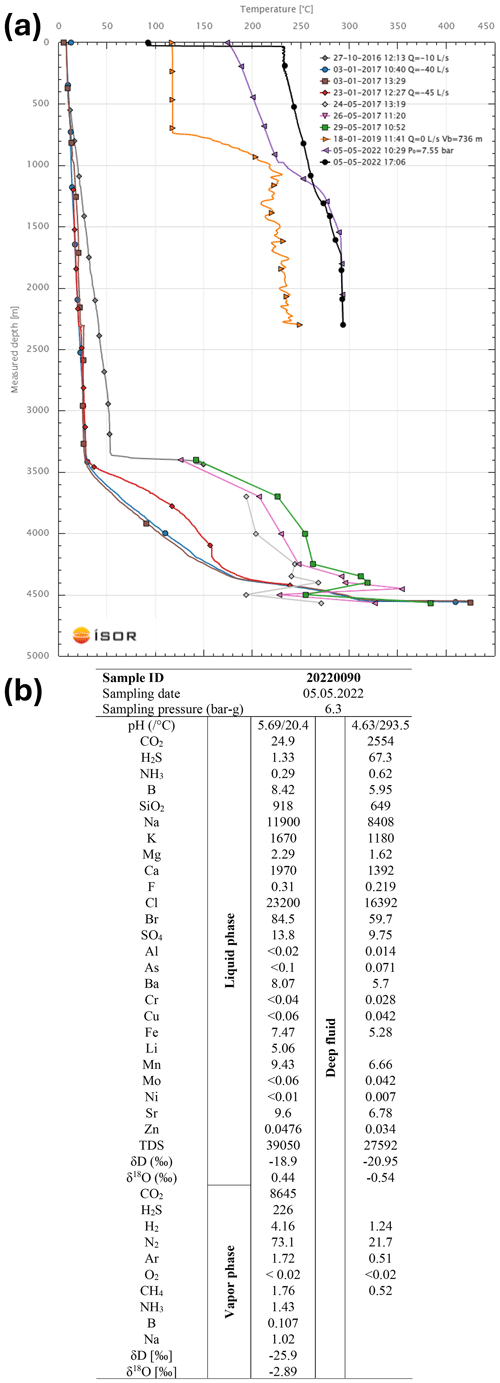
Figure 2Chosen T logs obtained during RN-15/IDDP-2 drilling and well testing, including the recent 2022 flow test (a). The raw analytical results given in (b) show pH, the isotopic composition and dissolved solids in mg kg−1 in the liquid phase and the isotopic composition and the gas contend in the vapor phase in mg kg−1 of steam. The calculated deep fluid composition (mg kg−1) at the quartz geothermometer is also shown in (b).
The bubble point of the deep fluid was calculated to be 82 bar-a compared to 71–73 bar-a before the IDDP-2 drilling. The deep fluid pH was calculated to be 4.63. The calculated liquid enthalpy using the discharge chemical composition at quartz temperature was calculated to be about 1310 kJ kg−1. For comparison the measured enthalpy during the flow test was 1127, 1104, and 609 kJ kg−1, respectively for step 1, 2, and 3. The highest gas content in vapor was measured in sample 20220094 at the lowest opening of 1.2 cm and it was equal to 1.83 wt % (Table 1).
5.1 RN-15 before drilling RN-15/IDDP-2
Well RN-15 was drilled in 2004. It was vertical, 2507 m deep, cased to 804 m. This well had an estimated reservoir temperature of 285 °C based on the temperature logs and deep chemical composition of the feeding aquifer (e.g., Galeczka and Óskarsson, 2019) located at 2395 m depth. The discharge enthalpy measured using tracer flow testing in 2016 was 1275 kJ kg−1 (Čypaitė et al., 2018) similar to the calculated liquid enthalpy of 1263 kJ kg−1 at an estimated reservoir temperature. In contrast to some other wells (e.g., RN-10, -11) a progressive reservoir boiling until 2009 caused by depressurization due to intensive geothermal production was not clearly seen based on the non-volatiles' concentrations and gas ratios evolution (Fig. 3; Galeczka and Óskarsson, 2019). The shifting of δD and δ18O towards the heavier isotopes until 2010, however, indicated aquifer boiling. Similar to RN-12 which is one of the deepest wells in the field, the concentrations of CO2, H2S, and H2 increased with time. At the same time the N2 concentration decreased. After 2009–2010, those concentrations and ratios stabilized or decreased indicating reservoir steady state in response to the geothermal production.
The decrease in Mg concentration with time until 2014 correlated with the increasing temperature calculated using quartz geothermometer. The B concentration in samples from this well was among the lowest measured in the Reykjanes production wells. The relatively low B, Na, K and Cl concentrations and a relative depletion in δD and δ18O compared to the other wells were explained by a recharge of less saline than seawater fluid into the aquifer feeding RN-15. Furthermore, a decrease in the non-volatile concentrations around 2009 suggested an increased inflow/recharge of less saline waters at that time. The decrease of some non-volatiles (Cl, Na, Ca, B) concentrations and shifting of the isotopic composition towards lighter isotopes after 2012 might have also been a result of re-injection into the well RN-33 in 2015. The tracer injected together with the re-injection mixture of freshwater, brine and condensate into RN-33 was measured in substantial amounts in RN-15.
5.2 Current chemical composition of RN-15/IDDP-2
The differences in the calculated deep composition of RN-15/IDDP-2 compared to the deep aquifer in 2004–2016 suggest that the fluid feeding this well changed somehow after its drilling, and testing. As can be seen in Figure 3, the concentration of SiO2 in the deep fluid based on the 2022 sample is 1.5 % higher compared to the average historical deep SiO2 concentration, suggesting slightly higher reservoir temperature compared to 2016. For comparison, the average quartz temperature calculated for the reservoir based on the samples collected in 2004–2016 was 291.3 °C with minimum of 275.2 °C in 2006 and maximum of 325.5 °C in 2010 (Óskarsson et al., 2015; Galeczka and Óskarsson, 2019). The former one most likely stems from insufficient discharge after drilling and the latter due to errors in sampling. In contrast, the other dissolved solutes concentrations are lower by 7 % for Na and Ca, 11 % for K, 8 % for Cl, 20 % for B, and 40% for SO4 compared to the average deep 2004–2016 concentrations and are in the low range of the Reykjanes deep fluid (Fig. 3). Note, that these concentrations in deep aquifer in RN-15/IDDP-2 have always been slightly lower compared to the other wells in Reykjanes.
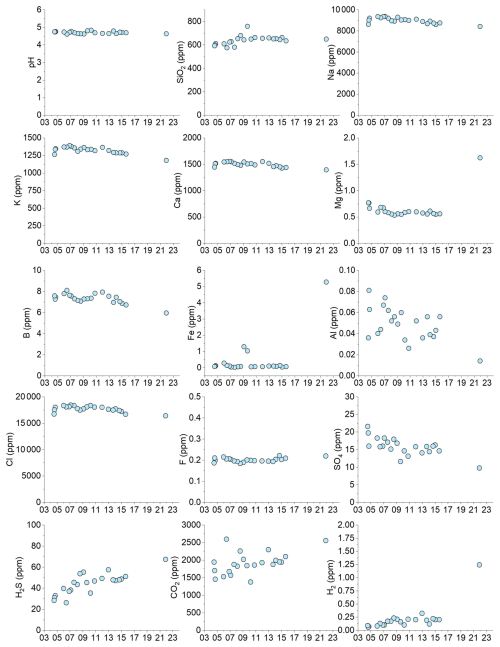
Figure 3The temporal evolution of the chemical composition of the deep fluid in RN-15/IDDP-2. The horizontal axis represents years. The last data-point on each plot depicts the composition calculated based on the sample collected during this study.
The Mg concentration is, however, higher compared to 2016 and it is in the upper range of Mg concentrations calculated for the Reykjanes reservoir. This concentration increase might be also due to higher stability of Mg-Cl species at higher temperature. The deep fluid pH of 4.63 is similar to the average pH of 4.71 calculated based on the samples collected from 2004 till 2016 (Fig. 3; Galeczka and Óskarsson, 2019). Note that the last production phase sample from RN-15 before drilling of the IDDP-2 was collected in 2016. The isotopic signature is heavier compared to the last sample collected from RN-15/IDDP-2, however, within the range of the deep Reykjanes fluid. For comparison, the groundwater in vicinity of the Reykjanes geothermal area has an isotopic composition of δD from −53 to −49 and δ18O from −7 to −8 ‰ (e.g., Galeczka and Óskarsson, 2019).
There is a substantial increase in volatiles content (CO2, H2S, H2, N2) in the deep fluid compared to 2004–2016 (Fig. 3). The concentrations of the dissolved gases are higher by 36 % for CO2, 56 % for H2S, 63 % for N2, factor of 3.5 for CH4 and a factor of 8 for H2 compared to the concentrations of these volatiles in the past. The CO2 and H2 concentrations in the deep fluid have never been as high as during the current flow test. The NH3 concentration is, however, lower by 62 %. The deep fluid is undersaturated with respect to calcite and at equilibrium with respect to anhydrite, similar to what was previously calculated for RN-15. The Fe concentration in the deep fluid based on the 2022 sample was relatively high, however, concentrations of > 2 ppm in the hottest production well, RN-10, have previously been observed and they were not associated with corrosion (e.g., Óskarsson et al., 2015). Contribution of Fe and H2 due to corrosion cannot, however, be excluded since the casing was damaged during the RN-15/IDDP-2 testing and therefore making it more fragile and therefore reactive (e.g., Oppong Boakye et al., 2022). The N2 concentration was higher than currently measured only a few times in the Reykjanes production history: in RN-22 in 2009, and during the drilling/testing of RN-15/IDDP-2 in 2017/2018 in RN-11, 12, 22, 28. Part of the N2 in the RN-15/IDDP-2 aquifer could originate from the air-saturated water that was injected into the well during drilling and testing in corroboration with lower concentration of major non-volatiles. The dilution of the Reykjanes aquifer in its NW-W part has previously been observed as a result of re-injection into RN-33 and RN-20b indicating its connection to the main production aquifer. The isotope signature does not, however, confirm an inflow of the groundwater that was partly used for testing and re-injection.
The slightly increased temperature and relatively high gas content comparing to 2016 in the fluid produced from RN-15/IDDP-2 suggest a presence of a deeper fluid (or its boiling products) that is sourced below the aquifer at 2300 m depth. The logs obtained during drilling and testing in 2016–2017 show a rapid increase in temperature below approximately 3400 m, indicating that most of the injected water entered the formation at that depth. In addition, these logs suggest a connection to the reservoir at about 4400 m depth, where supercritical conditions are expected. Based on the fluid chemistry and enthalpy of the fluid measured at surface, it was assumed that during the 2022 flow test, most of the fluid discharging from the well, was likely entering the wellbore at the depth of the casing damage at just below 2300 m, with a very limited inflow from the other feed zones. Connection to the reservoir at about 4400 m depth, at supercritical conditions was observed during logging in previous years (Fig. 2). The supercritical fluids can be characterized by elevated CO2, SO2, HCl, and HF concentrations if they originate from magmatic degassing. If they form due to conductive heat transfer from a magmatic intrusion to a subcritical fluid, they will have a similar volatile content as a subcritical reservoir fluid and low concentration of non-volatiles (Heřmanská et al., 2019). For example, the superheated vapour discharged from IDDP-1 in Krafla, had low concentrations of alkali and earth alkaline metals and other non-volatile elements but elevated concentrations of SiO2, HCl, HF and other constituents that are soluble in vapour at high temperature and pressure (Ármannsson et al., 2014; Heřmanská et al., 2019). Existence of a vapor rich supercritical phase dominated by water with CO2, H2S and H2 and a Fe-, K-, Cl-rich supercritical brine were confirmed in RN-15/IDDP-2 by analysis of fluid inclusions from a drill core collected from > 4500 m depth in the well (Bali et al., 2020). Modelling of a hypothetical fluid formed by mixing of 5 %–10 % of a vapour-rich supercritical phase such as that reported by Bali et al. (2020) with conventional geothermal fluid from Reykjanes after heating to 350 °C (Óskarsson, 2020), suggestes some of the characteristics observed in the sample from RN-15/IDDP-2, including lower concentrations of many major elements (Na, K, Ca, Cl) but higher concentrations of reactive gases (CO2, H2S, H2) and some minor elements (Fe, Mg, Mn, Zn). Therefore, a limited inflow of supercritical fluid cannot be excluded based on the current RN-15/IDDP-2 sample.
The fluid currently discharging from the RN-15/IDDP-2 well most likely originates mainly from a feed zone at just below 2300 m depth where the well casing is damaged. This feed zone was considered as the main production aquifer in the RN-15 well before IDDP-2 was drilled. Although the current calculated deep chemical composition is similar to the composition of the aquifer feeding RN-15 before 2016, higher concentrations of some of the constituents (e.g., volatiles and some metals) and gas content suggest inflow from feed zones located at greater depths and therefore at higher temperatures. Further investigation of the chemical composition of the well discharge is needed to quantify the contribution of various feed zones in the total discharge and to confirm if the signature of a supercritical fluid from 4400 m depth is present in this well.
No data sets were used in this article.
IG – writing the original draft, investigation, analysis, FÓ – validation, writing – review and editing, KG – resources, JJ – investigation, writing – review and editing.
Kiflom Gebrehiwot Mesfin works at HS Orka. The authors have no other competing interests to declare.
Publisher's note: Copernicus Publications remains neutral with regard to jurisdictional claims made in the text, published maps, institutional affiliations, or any other geographical representation in this paper. While Copernicus Publications makes every effort to include appropriate place names, the final responsibility lies with the authors.
This article is part of the special issue “European Geosciences Union General Assembly 2024, EGU Division Energy, Resources & Environment (ERE)”. It is a result of the EGU General Assembly 2024, Vienna, Austria, 14–19 April 2024.
The IDDP-2 was funded by HS Orka, Landsvirkjun, Reykjavik Energy and National Energy Authority, in Iceland, together with Equinor (former Statoil). The IDDP-2 also received funding from the EU H2020 (DEEPEGS grant no. 690771) for all parts of the operation, and ICDP and US NSF (grant No. 05076725) for spot coring and part of the related research. The authors would like to thank the editor Michael Kühn for handling this manuscript. Thomas Baumann and the other reviewer are greatly thanked for their constructive comments which improved the manuscript.
This paper was edited by Michael Kühn and reviewed by Thomas Baumann and one anonymous referee.
Arnórsson, S.: Major element chemistry of the geothermal sea-water at Reykjanes and Svartsengi, Iceland: Min. Mag., 42, 209–220, https://doi.org/10.1180/minmag.1978.042.322.07, 1978.
Arnórsson, S.: Isotopic and chemical techniques in geothermal exploration, development and use: sampling methods, data handling, interpretation, International Atomic Energy, Vienna, ISBN 92-0-101600-X, 2000.
Arnórsson, S., Sigurdsson, S., and Svavarsson, H.: The chemistry of geothermal waters in Iceland. I. Calculation of aqueous speciation from 0° to 370 °C, Geochim. Cosmochim. Act., 46, 1513–1532, https://doi.org/10.1016/0016-7037(82)90311-8, 1982.
Ármannsson, H., Fridriksson, T., Gudfinnsson, G.H., Ólafsson, M., Óskarsson, F., and Thorbjörnsson, D.: IDDP – The chemistry of the IDDP-01 well fluids in relation to the geochemistry of the Krafla geothermal system, Geothermics, 49, 66–75, https://doi.org/10.1016/j.geothermics.2013.08.005, 2014.
Bali, E., Aradi, L. E., Zierenberg, R., Diamond, L. W., Pettke, T., Szabó, Á., Guðfinnsson, G. H., Friðleifsson, G.Ó., and Szabó, C.: Geothermal energy and ore-forming potential of 600 °C mid-ocean-ridge hydrothermal fluids, Geol. 48, 1221–1225, https://doi.org/10.1130/G47791.1, 2020.
Bjarnason, J.Ö.: Manual for the chemical speciation program WATCH version 2.4. Iceland GeoSurvey, Accountant – Jupiter X Templates, https://en.isor.is/software/ (last access: 2 November 2024), 2010.
Čypaitė, V., Óskarsson, F., and Jónsson, S. S.: Reykjanes power plant. Tracer flow testing of production wells in June 2018. Iceland GeoSurvey, short report ÍSOR-18029, 2018.
Elders, W. A., Fridleifsson, G. Ó., and Albertsson, A.: Drilling into magma and the implications of the Iceland Deep Drilling Project (IDDP) for high-temperature geothermal systems worldwide, Geothermics, 49, 111–118, https://doi.org/10.1016/j.geothermics.2013.05.001, 2014.
E.T.: The Engineering ToolBox. Saturated Steam – Properties for Pressure in Bar, https://www.engineeringtoolbox.com/saturated-steam-properties-d_457.html (last access: 2 November 2024), 2003.
Freedman, J. E., Bird, D. K., Arnórsson, S., Fridriksson, Th., Elders, W. A., and Fridleifsson, G. Ó.: Hydrothermal Minerals Record CO2 Partial Pressure in the Reykjanes Geothermal System, Iceland, Am. J. Sci., 309, 788–833, https://doi.org/10.2475/09.2009.02, 2009.
Fournier, R. O. and Potter, R. W.: A revised and expanded silica (quartz) geothermometer. Bull., Geotherm. Resour. Counc. (Davis, Calif.), (United States) 11, 3–12, OSTI ID:5703508, 1982.
Friðleifsson, G. Ó. and Albertsson, A.: Deep geothermal drilling at Reykjanes Ridge: opportunity for an international collaboration, Proceedings of the World Geothermal Congress, Japan, May 28–June 10, 3701–3706, 2000.
Friðleifsson, G. Ó., Elders, W. A., Zierenberg, R. A., Stefánsson, A., Fowler, A. P. G., Weisenberger, T. B., Harðarson, B. S., and Mesfin, K. G.: The Iceland Deep Drilling Project 4.5 km deep well, IDDP-2, in the seawater-recharged Reykjanes geothermal field in SW Iceland has successfully reached its supercritical target, Sci. Dril., 23, 1–12, https://doi.org/10.5194/sd-23-1-2017, 2017.
Friðleifsson, G.Ó., Elders, W.A., Zierenberg, R.A., Fowler, A.P.G., Weisenberger, T.B., Mesfin, K.G., Sigurðsson, Ó., Níelsson, S., Einarsson, G., Óskarsson, F., Guðnason, E.Á., Tulinius, H., Hokstad, K., Benoit, G., Nono, F., Loggia, D., Parat, F., Cichy, S.B., Escobedo, D., and Mainprice, D.: The Iceland Deep Drilling Project at Reykjanes: Drilling into the root zone of a black smoker analogue, J. Volc. Geoth. Res. 391, 106435, https://doi.org/10.1016/j.jvolgeores.2018.08.013, 2020.
Galeczka, I. M. and Óskarsson, F.: Reykjanes production field. Geochemical monitoring in 2018, Iceland GeoSurvey, ÍSOR-2019/005, 2019.
Hardardóttir, V., Brown, K. L., Fridriksson, Th., Hedenquist, J. W., Hannington, M. D., and Thórhallsson, S.: Metals in deep liquid of the Reykjanes geothermal system, southwest Iceland: Implications for the composition of seafloor black smoker fluids, Geol., 37, 1103–1106, https://doi.org/10.1130/G30229A.1, 2009.
Hauksson, T., Markússon, S., Einarsson, K, Karlsdóttir, S. N., Einarsson, Á., Möller, A., and Sigmarsson, Th.: Pilot testing of handling the fluids from the IDDP-1 exploratory geothermal well, Krafla, N.E. Iceland, Geothermics, 49, 76–82, https://doi.org/10.1016/j.geothermics.2013.07.003, 2014.
Heřmanská, M., Kleine, B. I., and Stefánsson, A.: Supercritical Fluid Geochemistry in Geothermal Systems, Geofluids, 6023534, https://doi.org/10.1155/2019/6023534, 2019.
Jónsson, J. E. and Galeczka, I. M.: Reykjanes – Well RN-15/IDDP-2, Flow Testing in May 2022. Report prepared for HS Orka Ltd. ÍSOR-2022/033, 2022.
Khodayar, M., Björnsson, S., Guðnason, E.Á., Níelsson, S., Axelsson, G., and Hickson, C.: Tectonic Control of the Reykjanes Geothermal Field in the Oblique Rift of SW Iceland: From Regional to Reservoir Scales, Open J. Geol., 8, 333–382, https://doi.org/10.4236/ojg.2018.83021, 2018.
Ólafsson, M. and Ármannsson, H.: Collection of geothermal fluids for chemical analysis, Iceland GeoSurvey, ÍSOR-2006/016, 2006.
Oppong Boakye, G., Geambazu, L. E.; Ormsdottir, A. M., Gunnarsson, B. G., Csaki, I., Fanicchia, F., Kovalov, D., and Karlsdottir, S. N.: Microstructural Properties and Wear Resistance of Fe-Cr-Co-Ni-Mo-Based High Entropy Alloy Coatings Deposited with Different Coating Techniques. Appl. Sci., 12, 3156, https://doi.org/10.3390/app12063156, 2022.
Óskarsson, F.: Composition of reservoir fluids in well IDDP-2, Proceedings World Geothermal Congress 2020+1, Iceland, April–October, https://worldgeothermal.org/ (last access: 20 December 2024), 2021.
Óskarsson, F., Fridriksson, Th., and Thorbjörnsson, D.: Geochemical monitoring of the Reykjanes Geothermal Reservoir 2003-2013. Proceedings World Geothermal Congress 2015, Australia, Melbourne, 19–25 April, https://www.geothermal-energy.org/cpdb/record_detail_v1.php?id=23928 (last access: 20 December 2024), 2015.
Reinsch, T., Dobson, P., Asanuma, H., Huenges, E., Poletto, F., and Sanjuan, B.: Utilizing supercritical geothermal systems: a review of past ventures and ongoing research activities, Geotherm. Energy, 5, 16, https://doi.org/10.1186/s40517-017-0075-y, 2017.
Sæmundsson, K., Sigurgeirsson, M., and Friðleifsson, G.O.: Geology and structure of the Reykjanes volcanic system, Iceland, J. Vol. Geoth. Res. 391, 106501, https://doi.org/10.1016/j.jvolgeores.2018.11.022, 2020.
Sæther, S.: Estimating flow performance of IDDP-2/DEEPEGS well by introducing local injectivity indexes for different reservoir depths. Proceedings World Geothermal Congress 2020+1, Iceland, April–October, https://www.geothermal-energy.org/pdf/IGAstandard/WGC/2020/22045.pdf (last access: 20 December 2024), 2020.
Sigmundsson, F., Parks, M., Geirsson, H., Hooper, A., Drouin, V., Vogfjörd, K. S., Ófeigsson, B. G., Greiner, S. H. M., Yang, Y., Lanzi, C., De Pascale, G. P., Jónsdóttir, K., Hreinsdóttir, S., Tolpekin, V., Friðriksdóttir, H. M., Einarsson, P., and Barsotti, S.: Fracturing and tectonic stress drive ultrarapid magma flow into dikes, Science, 383, 1228–1235, https://doi.org/10.1126/science.adn2838, 2024.
Weisenberger, T. B., Harðarson, B., Mesfin, K. G., Einarsson, G. M., Nielsson, S., Zierenberg, R., and Friðleifsson G. O.: The Iceland Deep Drilling Project at Reykjanes – 4.5 km Deep Drilling into Supercritical Conditions. Proceedings, 44th Workshop on Geothermal Reservoir Engineering, Stanford University, 11–13 February , SGP-TR-214, https://pangea.stanford.edu/ERE/db/GeoConf/papers/SGW/2019/Weisenberger.pdf (last access: 20 December 2024), 2019.
Zarrouk, S. J. and McLean, K.: Geothermal Well Test Analysis. Academic Press. Fundamentals, Applications and Advanced Techniques, ISBN 9780128192665, 2019.
Zierenberg, R. A., Friðleifsson, G.Ó., Elders, W. A., Schiffman, P., and Fowler, A. P. G.: Active basalt alteration at supercritical conditions in a seawater-recharged hydrothermal system: IDDP-2 drill hole, Reykjanes, Iceland, Geochem. Geophys. Geosyst., 22, e2021GC009747, https://doi.org/10.1029/2021GC009747, 2021.