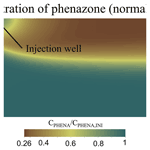
Influence of shallow geothermal energy on the behaviour of organic contaminants of emerging concern in urban aquifers
Estanislao Pujades
Laura Scheiber
Marc Teixidó
Rotman Criollo
Olha Nikolenko
Victor Vilarrasa
Enric Vázquez-Suñé
Anna Jurado
Urban aquifers are a valuable resource of freshwater for cities, however, their quality is degraded due to the presence of organic contaminants of emerging concern (CECs). The effects of organic CECs are largely unknown, but there is evidence that they pose a risk for human health, soil, plants and animals. Organic CECs are naturally degraded in aquifers and their degradation rates depend on the physico-chemical properties, i.e., redox conditions and groundwater temperature. Some anthropogenic activities, like low-enthalpy geothermal energy (LEGE), may modify subsurface physico-chemical conditions altering the behaviour of organic CECs. LEGE is a renewable and carbon-free energy that allows obtaining cooling and heating energy. The utilization of LEGE is currently growing and it is expected that in a near future the density of LEGE systems will increase. LEGE modifies the groundwater temperature and in some situations the redox state (i.e., if the dissolved oxygen increases when groundwater is returned to the aquifer as a result of a poorly design), thus, it is of paramount importance to determine the impact of LEGE related activities on the behaviour of organic CECs. The behaviour of organic CECs under the influence of LEGE is investigated by means of thermo-hydro-chemical numerical modelling. Simulation output shows that LEGE activities have the potential to modify the degradation rates of organic CECs, and thus, their concentrations in aquifers. In the simulated scenario, the concentration of the chosen CEC decreases by the 77 % at the downgradient boundary of the model. The results of this study have significant implications for predicting the behaviour of organic CECs in urban aquifers and suggest specific changes in the design of LEGE facilities aiming to improve the quality of urban groundwater by boosting in-situ attenuation mechanisms.
- Article
(832 KB) - Full-text XML
- BibTeX
- EndNote
In the current context of climate change and urban sprawl, it is necessary to take advantage of urban groundwater bodies to cover the increasing water demand. However, urban groundwater bodies are usually highly polluted by a wide range of anthropogenic contaminants, such as organic contaminants of emerging concern (CECs) and their transformation products (TPs) (Lapworth et al., 2019). Organic CECs comprise natural and anthropogenic substances of organic nature such as pharmaceuticals and personal care products. These pollutants, which are not removed in wastewater treatment plants, reach groundwater bodies through different recharge sources such as water leakage from sewer and septic systems, seepage from rivers or artificial recharge activities (Jurado et al., 2020). Organic CECs pose human health and ecological risks (Arnold et al., 2014; Tran et al., 2013) and for this reason they deserve being investigated to assure the safe usage of urban groundwater resources.
Organic CECs are degraded or transformed in aquifers by microbial activity (Greskowiak et al., 2017), which is reflected in lower concentrations found in aquifers in comparison to rivers (Jurado et al., 2021). Physico-chemical conditions of aquifers play an important role in the degradation of organic CECs since it is a redox-dependent process (Burke et al., 2014) that varies with temperature (Burke et al., 2017).
Groundwater temperature is not a commonly considered parameter when investigating the degradation of organic CECs since it is almost constant during the whole year, except close of surface water bodies that may influence the groundwater temperature. However, it can be altered due to anthropogenic activities, like the use of the subsurface for energetic purposes by means of low-enthalpy geothermal energy (LEGE). LEGE is a carbon-free and renewable energy that is used for cooling and heating buildings and has a great potential to supply a large portion of the heating and cooling demand. In fact, its use is growing continuously in recent years (García-Gil et al., 2020). LEGE provides cooling and heating energy by exchanging the temperature of groundwater with the temperature of buildings through heat exchangers (Cui et al., 2014). Cooling energy is obtained by transferring the building heat to groundwater, resulting in an increase in groundwater temperature. In contrast, for heating, the groundwater heat is transferred to the building, decreasing the temperature of groundwater. Consequently, as a result of LEGE activities, the groundwater temperature varies.
The hypothesis of this study is that groundwater temperature variations, produced by LEGE facilities, impact the behaviour of organic CECs by increasing or decreasing their degradation/transformation rates. The investigation of this hypothesis is not only important to better understand the behaviour of organic CECs, but also to evaluate the possibility of designing LEGE facilities to improve the quality of groundwater by enhancing the degradation capacity of aquifers against organic CECs. This hypothesis is deduced from previous investigations in the context of river bank filtration and polluted aquifers by chlorinated organic compounds (COCs). On the one hand, investigations developed in the context of river bank filtration show that the seasonal variations of the river temperature modify the evolution of organic CECs in the aquifer (Barkow et al., 2021). On the other hand, groundwater temperature variations, induced by LEGE facilities, seem to improve the dichlorination capacity of aquifers polluted by industrial compounds (Hoekstra et al., 2020; Pellegrini et al., 2019). Despite of these relevant investigations, the influence of LEGE on the behaviour of organic CECs at the aquifer scale remains to be investigated.
Thus, our main objective is to assess the potential impact of LEGE on the behaviour of organic CECs present in groundwater bodies. The problem is addressed numerically with a synthetic thermo-hydro-chemical numerical model that simulates an aquifer polluted by phenazone and in which a LEGE facility used for cooling purposes is implemented.
2.1 Problem statement
The problem consists in a 20 m thick fully-saturated and homogeneous sandy aquifer made of unconsolidated materials. The aquifer has a length of 2000 m and a width of 1000 m (Fig. 1a).

Figure 1(a) General view of the problem. (b) Detailed view of the modelled area including the boundary conditions (BC).
The LEGE facility, which is located in the middle of the considered aquifer, is of the groundwater heat pump (GWHP) type (Lee et al., 2006) and consists of two wells, the production and the injection well. The production well is located upgradient while the injection one is located downgradient. It is assumed a negligible conductive heat loss to overlying or underlying strata (i.e., groundwater temperature is not dissipated thought surrounding strata). Pumping and injection rates (Q) are assumed to be constant and equal to 432 m3 d−1 (i.e., 5 L s−1). The injection rate has been chosen according with the transmissivity to slightly modify the hydraulic gradient and minimize the risk of thermal breakthrough. The wells are separated 290 m to avoid thermal breakthrough during the simulated time. The minimum distance between wells to avoid thermal breakthrough has been computed according to Banks (2009) considering hydraulic parameters shown in Table 1 and a hydraulic gradient of 0.005.
Table 1Aquifer parameters. Values are chosen according to those typical for homogenous sandy aquifers (K: Domenico and Schwartz, 1998; θ: Woessner and Poeter, 2020; DL: Schulze-Makuch, 2005). DT is chosen ten times lower than DL, which is common practice (Zech et al., 2019) and Dm is chosen according to the reference values from the German Engineer Association guidelines for thermal use of the underground (VDI, 2019).
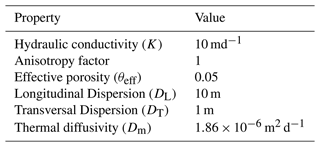
It is assumed that the simulated LEGE facility is only used for cooling and the obtained cooling potential is constant. Though LEGE facilities are commonly used for cooling and heating purposes depending on the season, they can be also used only for cooling. Continuous cooling energy can be used by neighbourhood factories or other infrastructures that need to be continuously refrigerated, such as data centres containing information technology equipment or high-performance computing systems (Zurmuhl et al., 2019). We consider that the temperature of the pumped groundwater is increased by 15 ∘C to obtain cooling energy. Then, considering a groundwater temperature under unperturbed conditions of 20 ∘C and that water is injected at 35 ∘C, the thermal potential (PGW) of the facility is 313 800 W, i.e., 225 936 kWh per month. PGW is computed as follows:
where SVCwat is the is the volumetric heat capacity of water at 20 ∘C (4180 Jkg−1 K−1) and ΔT is the temperature difference between the wells.
It is considered that the modelled aquifer is polluted by phenazone, which is homogenously distributed at a concentration of mol L−1. Phenazone is an analgesic drug that is commonly reported in urban aquifers (Reddersen et al., 2002; Jurado et al., 2021). Its degradation is oxygen and temperature dependent (Greskowiak et al., 2006) and its behaviour is similar to other redox and temperature dependent organic CECs. The aquifer is characterized with aerobic conditions and has a moderate value of dissolved oxygen (2 mg L−1). This value of dissolved oxygen is chosen according with reported data from Barcelona's aquifers (Jurado et al., 2013).
2.2 Numerical model
The numerical model is developed with the code PHT3D (Prommer et al., 2003). Theoretically, if the distance between the production and injection wells is correctly chosen, the hydraulic head at the middle of the domain should be constant and equal to that under initial conditions. Thus, taking advantage of the symmetry of the problem, only 1/4 of the problem is modelled (Fig. 1b). The simulated domain covers, in the flow direction, from the centre of the aquifer to the downgradient boundary, and, in the perpendicular direction to the flow, from the centre until the southern boundary. Twenty years is the total simulated time and the time steps are of 30 and 1 d for the flow and reactive transport problems, respectively. The difference between flow and transport time steps are due to convergence issues. Smaller time steps for the flow are not required since the pumping and injection rates are constant, however, short time steps are used for the transport to avoid convergence problems. A regular mesh consisting of one layer divided into 5000 elements is used.
Two types of flow boundary conditions (BCs) are implemented. Specified head BCs are implemented at the upgradient and downgradient boundaries to reach a hydraulic gradient under unperturbed conditions of 0.005, while specified flow BCs are adopted in the injection well. No-flow BCs are assumed in the other boundaries. Concerning the transport BCs, concentrations of mol L−1 of phenazone and 2 mg L−1 of oxygen (O2) are prescribed to the upgradient boundary and in the injection well. The concentration of phenazone is in the same range of magnitude than reported data in previous works (Barkow et al., 2021). In addition, the required concentration of phenazone in the whole domain is reached by implementing a constant input mass of phenazone of mg d−1 per square meter. This BC represents the mechanisms driving the recharge of phenazone into groundwater since the recharge of organic CECs is a diffuse process (Drew and Hotzl, 1999; Wolf et al., 2012).
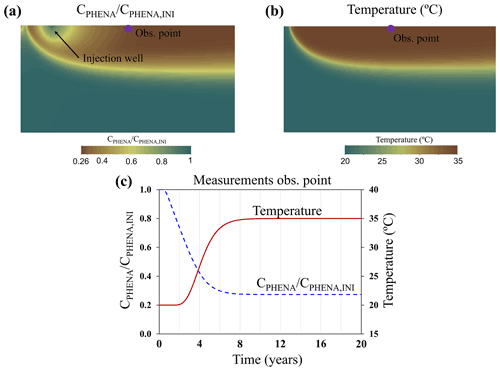
Figure 2Distribution of normalized concentration of phenazone (a) and groundwater temperature (b) at the end of the simulated period (i.e., 20 years). (c) Evolution of normalized concentration of phenazone (blue dashed line) and groundwater temperature (red continuous line) at the observation point located 500 m away from the injection well in the downgradient direction.
The degradation rate of phenazone (rPhena) is modelled considering Monod kinetics (Lu et al., 1999; Greskowiak et al., 2006) as follows:
where CPhena is the concentration of phenazone, is the maximum degradation rate constant of phenazone, is the Monod half-saturation constant of phenazone, is O2 concentration, and fT is a temperature-dependent function. Equation (2) approximates the degradation/transformation of phenazone as a 1st order degradation. In addition, it includes a term accounting for the concentration of O2, which is needed because the degradation rate of phenazone decreases with low concentration of O2. Finally, the impact of groundwater temperature is considering by implementing the additional factor fT. A normalized form of the Arrhenius equation is used to define fT as:
where A is a pre-exponential factor, EA is the activation energy, R is the gas constant, T the temperature in Kelvin and β is a factor to normalize the equation to 1 when the water temperature ranges between 35 and 40 ∘C. The required parameters to apply Eqs. (2) and (3) have been derived from bibliography and are presented in Table 2. A and EA are derived from Barkow et al. (2021), and λMAX and from Greskowiak et al. (2006).
Figure 2 shows the concentration of phenazone normalized by its initial concentration (A) after 20 years of simulation, the distribution of groundwater temperature (B) after 20 years of simulation and the evolution of phenazone (dashed blue line) and groundwater temperature (red continuous line) in an observation point located 500 m away from the injection well (C). Groundwater temperature increases up to 35 ∘C around the injection well and on the downgradient side. As a result, the degradation rate of phenazone in the area with high groundwater temperature increases according to Eqs. (2) and (3). The concentration of phenazone in the area affected by the LEGE facility decreases by 77 % in comparison to the initial concentration that does not vary far from the injection point where the aquifer is not affected by the injected water. Unlike the distribution of groundwater temperature, an area with high concentration of phenazone is observed around the injection well. This high concentration is the result of injecting the groundwater pumped in the production well, where the concentration of phenazone is high. In the top left corner of the model, it is possible to observe that the concentration of phenazone decreases. This occurs because in this corner, groundwater is not flowing as a result of the head distribution, and thus, groundwater with high concentration of phenazone does not inflow the model through this area. In addition, despite it is not observed in the figure because of colour bar limitations, the temperature is a little bit higher than that under unperturbed conditions that increases the degradation rate of phenazone. The measurements at the observation point (Fig. 2c) show how the concentration of phenazone decreases and the temperature increases during the simulated period. The concentration of phenazone starts to decrease before that the hot groundwater reaches the observation point. This behaviour is the consequence of the higher retardation factor for the heat transport than for phenazone transport. Note that no retardation factor was applied to phenazone because its sorption can be considered negligible (Greskowiak et al., 2006).
Our results are complementary to the conclusions of Hartog (2011), who assessed the influence of groundwater temperature induced by geothermal energy on groundwater quality. Hartog (2011) concluded that the impact of the temperature is relatively low if groundwater temperature does not exceed 25 ∘C. Here we probe that, certainly, the groundwater quality is substantially improved by reaching 35 ∘C around the injection well. Despite this is the first work analysing the impact of geothermal energy on organic CECs, other authors have assessed the behaviour of chlorinated compounds under the influence of geothermal facilities obtaining similar results. Among others, Ni et al. (2016) demonstrated through laboratory experiments that the degradation velocity increases (up to 13 times in their experiments) in comparison with natural conditions by the high groundwater temperature occurred around geothermal facilities. Beyer et al. (2016) also reached the same conclusions but in base of a numerical model. However, unlike us, Beyer et al. (2016) considered a geothermal facility of the closed-loop type where heat exchangers are introduced into the ground and groundwater is not pumped. This suggests that the concentration of organic CECs would also decrease under the influence of a geothermal facility of the closed-loop type.
This paper aims at investigating whether the thermal impact generated by LEGE facilities in aquifers can modify the behaviour of organic CECs. The synthetic numerical model considers the presence of phenazone, which is commonly reported in urban aquifers, and a LEGE system of the GWHP type that is used only for cooling purposes. A synthetic numerical model is used to allow the generalisation of the results. Obviously, site-specific numerical models will be needed when quantifying the influence of LEGE on CECs at real cases. The main conclusions that can be drawn from the results are:
-
The thermal impact produced by LEGE facilities on aquifers can considerably modify the behaviour of organic CECs. Thus, it is needed to consider the influence of these facilities when investigating the fate and evolution of organic CECs in groundwater bodies, especially in urban areas, where LEGE are expected to grow significantly in the coming years.
-
This investigation only considers a LEGE facility used for cooling. Thus, additional studies to investigate the behaviour of organic CECs under the influence of a LEGE facility used for heating and cooling will be needed. Theoretically, the degradation rate should decrease when using LEGE for heating. However, the exponential relation between degradation rate and temperature suggests that, overall, LEGE facilities used for heating and cooling will also enhance the degradation capacity of aquifers against organic CECs.
-
Simulation results suggest the possibility of designing LEGE facilities specifically to increase the degradation capacity of aquifers against organic contaminants which, consequently, improve the groundwater quality. This possibility worths to be deeply investigated in future research.
Data relative to the results and input files for the numerical model are available at https://doi.org/10.17605/OSF.IO/JXEAW (Pujades, 2022).
EP wrote the original draft of the paper and obtained the needed funding. EP, VV and AJ contributed to the conceptualization of the investigation. EP, MT, VV and AJ defined the methodology to reach the objectives. LS, MT, RC, ON, EVS, VV, AJ contributed to writing – review and editing the paper.
At least one of the (co-)authors is a guest member of the editorial board of Advances in Geosciences for the special issue “Quality and quantity issues in urban hydrogeology (EGU2022 HS8.2.8 session)”. The peer-review process was guided by an independent editor, and the authors also have no other competing interests to declare.
Publisher's note: Copernicus Publications remains neutral with regard to jurisdictional claims in published maps and institutional affiliations.
This article is part of the special issue “Quality and quantity issues in urban hydrogeology (EGU2022 HS8.2.8 session)”. It is a result of the EGU General Assembly 2022, Vienna, Austria, 23–27 May 2022.
Estanislao Pujades, Laura Scheiber and Rotman Criollo would like to thank the Ibero-American Programme of Science and technology for development (CYTED-Programa de ciencia y tecnologia para el desarrollo) under project 719RT0585. Rotman Criollo also thanks the support from the Balearic Island Government through the Margalida Comas postdoctoral fellowship programme (grant no. PD/036/2020). Finally, Victor Vilarrasa acknowledges support from the Agencia Estatal de Investigación (10.13039/501100011033), Spanish Ministry of Science and Innovation through the grant HydroPore (grant no. PID2019-106887GB-C32).
This research has been supported by the Barcelona city council through the Award for Scientific Research into Urban Challenges in the City of Barcelona 2020 (20S08708) and by the grants PID2021-128995OA-I00 funded by MCIN/AEI/10.13039/501100011033T and FEDER “one way to make Europe”, CEX2018-000794-S funded by MCIN/AEI/10.13039/501100011033 and RYC2020-029225-I funded by MCIN/AEI/10.13039/501100011033 and “ESF Investing in your future”.
We acknowledge support of the publication fee by the CSIC Open Access Publication Support Initiative through its Unit of Information Resources for Research (URICI).
This paper was edited by Miao Jing and reviewed by Oluwaseun Olabode, Laura Balzani, and Jannis Epting.
Arnold, K. E., Brown, A. R., Ankley, G. T., and Sumpter, J. P.: Medicating the environment: assessing risks of pharmaceuticals to wildlife and ecosystems, Philos. Trans. Roy. Soc. B, 369, 20130569, https://doi.org/10.1098/rstb.2013.0569, 2014.
Banks, D.: Thermogeological assessment of open loop well doublet schemes: an analytical approach, Proc. 27th Annual Conference of the Irish Group of the International Association of Hydrogeologists, 24–25 April 2007, Tullamore, County Offaly, Ireland, 1149–1155, 2009.
Barkow, I. S., Oswald, S. E., Lensing, H.-J., and Munz, M.: Seasonal dynamics modifies fate of oxygen, nitrate, and organic micropollutants during bank filtration – temperature-dependent reactive transport modeling of field data, Environ. Sci. Pollut. Res., 28, 9682–9700, https://doi.org/10.1007/s11356-020-11002-9, 2021.
Beyer, C., Popp, S., and Bauer, S.: Simulation of temperature effects on groundwater flow, contaminant dissolution, transport and biodegradation due to shallow geothermal use, Environ. Earth Sci., 75, 1244, https://doi.org/10.1007/s12665-016-5976-8, 2016.
Burke, V., Greskowiak, J., Asmuß, T., Bremermann, R., Taute, T., and Massmann, G.: Temperature dependent redox zonation and attenuation of wastewater-derived organic micropollutants in the hyporheic zone, Sci. Total Environ., 482–483, 53–61, https://doi.org/10.1016/j.scitotenv.2014.02.098, 2014.
Burke, V., Greskowiak, J., Grünenbaum, N., and Massmann, G.: Redox and Temperature Dependent Attenuation of Twenty Organic Micropollutants – A Systematic Column Study, Water Environ. Res., 89, 155–167, https://doi.org/10.2175/106143016X14609975746000, 2017.
Cui, P., Man, Y., and Fang, Z.: Geothermal Heat Pumps, in: Handbook of Clean Energy Systems, edited by: Yan, J., 1–22, https://doi.org/10.1002/9781118991978.hces041, 2014.
Domenico, P. A. and Schwartz, F. W.: Physical and Chemical Hydrogeology, John Wiley and Sons, Inc., New York, NY, USA, 506 pp., 1998.
Drew, D. and Hötzl, H. (Eds.): Karst Hydrogeology and Human Activities: Impacts, Consequences and Implications, 1st edn., Routledge, https://doi.org/10.1201/9780203749692, 1999.
García-Gil, A., Abesser, C., Gasco Cavero, S., Marazuela, M. Á., Mateo Lázaro, J., Vázquez-Suñé, E., Hughes, A. G., and Mejías Moreno, M.: Defining the exploitation patterns of groundwater heat pump systems, Sci. Total Environ., 710, 136425, https://doi.org/10.1016/j.scitotenv.2019.136425, 2020.
Greskowiak, J., Prommer, H., Massmann, G., and Nützmann, G.: Modeling Seasonal Redox Dynamics and the Corresponding Fate of the Pharmaceutical Residue Phenazone During Artificial Recharge of Groundwater, Environ. Sci. Technol., 40, 6615–6621, https://doi.org/10.1021/es052506t, 2006a.
Greskowiak, J., Hamann, E., Burke, V., and Massmann, G.: The uncertainty of biodegradation rate constants of emerging organic compounds in soil and groundwater – A compilation of literature values for 82 substances, Water Res., 126, 122–133, https://doi.org/10.1016/j.watres.2017.09.017, 2017.
Hartog, N.: Anticipated temperature effects on biogeochemical reaction rates in seasonal Aquifer Thermal Energy Storage (ATES) systems: an evaluation using the Arrhenius equation, in: 1e Nationaal Congres Bodemenergie, 13–14 October 2011, Utrecht, the Netherlands, 2011.
Hoekstra, N., Pellegrini, M., Bloemendal, M., Spaak, G., Andreu Gallego, A., Rodriguez Comins, J., Grotenhuis, T., Picone, S., Murrell, A. J., Steeman, H. J., Verrone, A., Doornenbal, P., Christophersen, M., Bennedsen, L., Henssen, M., Moinier, S., and Saccani, C.: Increasing market opportunities for renewable energy technologies with innovations in aquifer thermal energy storage, Sci. Total Environ., 709, 136142, https://doi.org/10.1016/j.scitotenv.2019.136142, 2020.
Jurado, A., Vàzquez-Suñé, E., Soler, A., Tubau, I., Carrera, J., Pujades, E., and Anson, I.: Application of multi-isotope data (O, D, C and S) to quantify redox processes in urban groundwater, Appl. Geochem., 34, 114–125, https://doi.org/10.1016/j.apgeochem.2013.02.018, 2013.
Jurado, A., Margareto, A., Pujades, E., Vázquez-Suñé, E., and Diaz-Cruz, M. S.: Fate and risk assessment of sulfonamides and metabolites in urban groundwater, Environ. Pollut., 267, 115480, https://doi.org/10.1016/j.envpol.2020.115480, 2020.
Jurado, A., Vázquez-Suñé, E., and Pujades, E.: Urban Groundwater Contamination by Non-Steroidal Anti-Inflammatory Drugs, Water, 13, 720, https://doi.org/10.3390/w13050720, 2021.
Lapworth, D. J., Lopez, B., Laabs, V., Kozel, R., Wolter, R., Ward, R., Amelin, E. V., Besien, T., Claessens, J., Delloye, F., Ferretti, E., and Grath, J.: Developing a groundwater watch list for substances of emerging concern: a European perspective, Environ. Res. Lett., 14, 035004, https://doi.org/10.1088/1748-9326/aaf4d7, 2019.
Lee, J.-Y., Won, J.-H., and Hahn, J.-S.: Evaluation of hydrogeologic conditions for groundwater heat pumps: analysis with data from national groundwater monitoring stations, Geosci. J., 10, 91, https://doi.org/10.1007/BF02910336, 2006.
Lu, G., Clement, T. P., Zheng, C., and Wiedemeier, T. H.: Natural Attenuation of BTEX Compounds: Model Development and Field-Scale Application, Groundwater, 37, 707–717, https://doi.org/10.1111/j.1745-6584.1999.tb01163.x, 1999.
Ni, Z., van Gaans, P., Smit, M., Rijnaarts, H., and Grotenhuis, T.: Combination of aquifer thermal energy storage and enhanced bioremediation: resilience of reductive dechlorination to redox changes, Appl. Microbiol. Biotechnol., 100, 3767–3780, https://doi.org/10.1007/s00253-015-7241-6, 2016.
Pellegrini, M., Bloemendal, M., Hoekstra, N., Spaak, G., Andreu Gallego, A., Rodriguez Comins, J., Grotenhuis, T., Picone, S., Murrell, A. J., and Steeman, H. J.: Low carbon heating and cooling by combining various technologies with Aquifer Thermal Energy Storage, Sci. Total Environ., 665, 1–10, https://doi.org/10.1016/j.scitotenv.2019.01.135, 2019.
Prommer, H., Barry, D. A., and Zheng, C.: MODFLOW/MT3DMS-Based Reactive Multicomponent Transport Modeling, Groundwater, 41, 247–257, https://doi.org/10.1111/j.1745-6584.2003.tb02588.x, 2003.
Pujades, E.: Influence of shallow geothermal energy on phenazone, OSF [data set], https://doi.org/10.17605/OSF.IO/JXEAW, 2022.
Reddersen, K., Heberer, T., and Dünnbier, U.: Identification and significance of phenazone drugs and their metabolites in ground- and drinking water, Chemosphere, 49, 539–544, https://doi.org/10.1016/S0045-6535(02)00387-9, 2002.
Schulze-Makuch, D.: Longitudinal dispersivity data and implications for scaling behavior, Groundwater, 43, 443–456, https://doi.org/10.1111/j.1745-6584.2005.0051.x, 2005.
Tran, N. H., Urase, T., Ngo, H. H., Hu, J., and Ong, S. L.: Insight into metabolic and cometabolic activities of autotrophic and heterotrophic microorganisms in the biodegradation of emerging trace organic contaminants, Bioresour. Technol., 146, 721–731, https://doi.org/10.1016/j.biortech.2013.07.083, 2013.
VDI: VDI 4640 Blatt 2 – Thermische Nutzung des Untergrunds – Erdgekoppelte Wärmepumpenanlagen, Verein Deutscher Ingenieure, https://www.vdi.de/richtlinien/details/vdi-4640-blatt-2-thermische-nutzung-des-untergrunds-erdgekoppelte-waermepumpenanlagen (last access: 10 August 2022), 2019.
Woessner, W. and Poeter, E.: Hydrogeologic Properties of Earth Materials and Principles of Groundwater Flow, The Groundwater Project, Guelph, Ontario, Canada, 205 pp., ISBN 978-1-7770541-2-0, 2020.
Wolf, L., Zwiener, C., and Zemann, M.: Tracking artificial sweeteners and pharmaceuticals introduced into urban groundwater by leaking sewer networks, Sci. Total Environ., 430, 8–19, https://doi.org/10.1016/j.scitotenv.2012.04.059, 2012.
Zech, A., Attinger, S., Bellin, A., Cvetkovic, V., Dietrich, P., Fiori, A., Teutsch, G., and Dagan, G.: A Critical Analysis of Transverse Dispersivity Field Data, Groundwater, 57, 632–639, https://doi.org/10.1111/gwat.12838, 2019.
Zurmuhl, D. P., Lukawski, M. Z., Aguirre, G. A., Law, W. R., Schnaars, G. P., Beckers, K. F., Anderson, C. L., and Tester, J. W.: Hybrid geothermal heat pumps for cooling telecommunications data centers, Energy Build., 188–189, 120–128, https://doi.org/10.1016/j.enbuild.2019.01.042, 2019.